Biotechnology and Tissue Engineering Insights

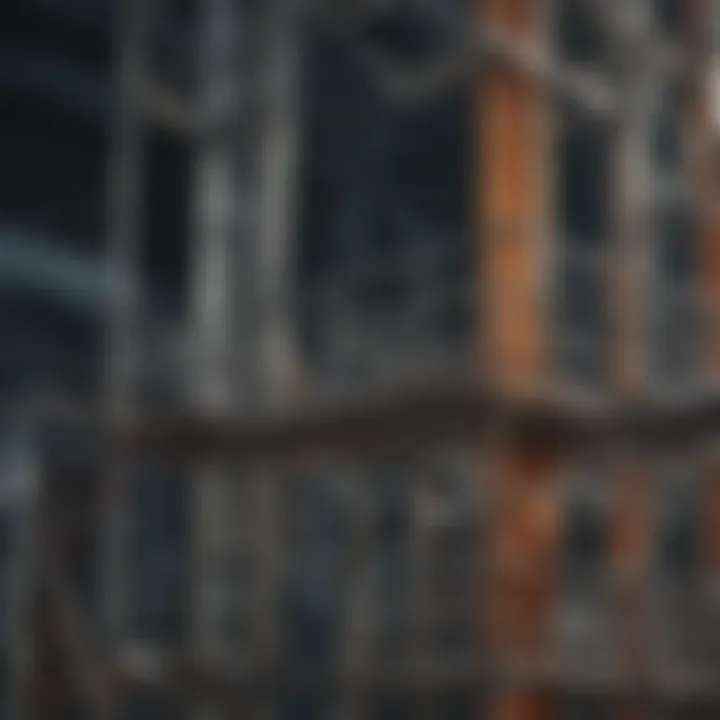
Intro
Biotechnology stands as one of the most transformative fields of science today, bridging gaps between biology, genetics, medicine, and engineering. Within this dynamic domain, tissue engineering emerges as a critical subset, aiming to recreate functional biological tissues that can replace or repair damaged ones. This intertwining of disciplines not only challenges our understanding of human biology but also paves the way for innovative treatments in regenerative medicine.
Tissue engineering relies on an intricate dance of various scientific methodologies, including innovative cell culture techniques, biomaterials, and scaffold design. As we dissect this subject, it is crucial to appreciate how these elements work in concert to create viable, living tissues. Every aspect of this field depends on a robust ethical framework, especially as the potential applications become both groundbreaking and ethically charged.
In the sections that follow, we will explore the foundational principles of biotechnology and tissue engineering, examining their historical evolution, key concepts, and contemporary methodologies, while also addressing the ethical landscape that surrounds this promising field.
Prelims to Biotechnology
Biotechnology merges biology with technology, leading to innovations that touch almost every aspect of our lives. With applications ranging from agriculture to medicine, its significance cannot be overstated, particularly in areas such as tissue engineering. In this article, the spotlight is on how these biotechnological principles are applied to create artificial organs and tissues, enhancing the landscape of regenerative medicine.
Definition and Scope
At its core, biotechnology refers to the utilization of living organisms or their components to develop products and processes that improve our quality of life. The scope of biotechnology extends widely: it encompasses genetic engineering, molecular biology, biochemistry, and beyond. This multidisciplinary nature allows for a combination of methods and approaches that can generate new tools and therapies, giving rise to cutting-edge solutions in health care, environmental management, and food production.
For instance, techniques such as CRISPR (Clustered Regularly Interspaced Short Palindromic Repeats) allow for precise editing of genes, which can lead to breakthroughs in treating genetic disorders. This highlights not just the innovative capacity of biotechnology, but also its ability to address complex challenges.
Historical Context
The roots of biotechnology can be traced back thousands of years, though its modern iteration gained momentum in the 20th century. The fermentation processes used by ancient civilizations to make beer and bread are early examples of biotechnology at work. However, fresh waves of discoveries in microbiology and genetics during the last century catalyzed a shift towards more advanced applications.
The development of insulin from recombinant DNA technology in the 1980s marks a pivotal moment. It showcased how far biotechnology could go in solving real-world health issues. Today, advancements continue at a brisk pace, interwoven with ethics and societal concerns, pressing the importance of regulation and responsible science in biotechnology practices.
Current Trends in Biotechnology
Present-day biotechnology is awash with trends that showcase its dynamic nature. Key among these is the rise of synthetic biology, which combines engineering principles with biological systems to create novel organisms or biological devices. By reprogramming these biological circuits, scientists aim to produce useful substances, from biofuels to pharmaceuticals.
Moreover, personalized medicine is becoming increasingly prominent as biotechnological advances allow for therapies tailored to an individual’s genetic makeup. This shift presents unique benefits, such as enhanced efficacy and reduced side effects, but also raises questions about privacy and data usage. Some other notable current trends include:
- Gene therapy: Aiming to treat or prevent diseases by inserting genes into a patient’s cells.
- Biomanufacturing: Utilizing organisms to produce compounds in environmentally sustainable ways.
- Biodegradable plastics: Developing new materials that can break down naturally, addressing plastic pollution.
Biotechnology isn’t just about science; it’s about reshaping our future, addressing societal challenges, and paving the way for sustainable development.
Biotechnology isn’t just about science; it’s about reshaping our future, addressing societal challenges, and paving the way for sustainable development.
In summary, the intersection of biotechnology and tissue engineering offers a roadmap into the future of medicine and environmental sustainability. As we delve deeper into tissue engineering, it becomes clear that understanding the fundamentals of biotechnology is key to unlocking its immense potential.
Understanding Tissue Engineering
The realm of tissue engineering bridges the ever-evolving fields of biology, engineering, and medicine. This discipline holds immense potential for addressing some of the most pressing challenges faced in healthcare today. The significance of understanding tissue engineering lies in its promise to replace, repair, and regenerate damaged tissues, fundamentally reshaping how we approach medical treatment and recovery.
Definition and Key Concepts
At its core, tissue engineering is the confluence of biology and engineering aimed at constructing functional biological tissues. The fundamental idea is to combine scaffolding materials, biological cells, and bioactive molecules, creating an environment conducive for cells to grow and function. These combinations are designed to replicate the structure and function of natural tissues, allowing for the development of tissues that can integrate seamlessly into the human body.
Key concepts in tissue engineering include:
- Scaffolds: Three-dimensional structures designed to support cell attachment and tissue development. Scaffolds can be made from either natural or synthetic materials, and their design can significantly influence the outcome of the engineered tissue.
- Cell Sources: Identifying appropriate cells is critical; these can be stem cells, differentiated cells, or even cells harvested from the patient's own body to reduce rejection risks.
- Biocompatibility: It's essential that the materials used do not elicit an adverse immune response. Materials should promote cell adhesion and growth while remaining inert.
This combination of elements ensures that engineered tissues can fulfill their biological roles within the body.
Objectives of Tissue Engineering
Understanding the objectives of tissue engineering is crucial for grasping its importance in modern medicine. Here are some primary aims:
- Regeneration: The primary goal is to restore or replace lost or damaged tissue function. This can include regenerating skin for burns, repairing cartilage for joint function, or even reconstructing organs.
- Reduction of Donor Dependency: By cultivating tissues in laboratories, we can minimize patients' reliance on donor organs, which is always in short supply.
- Personalized Medicine: Advances in tissue engineering raise the potential for creating patient-specific tissues, which can offer better compatibility and reduced rejection rates during transplantation.
- Drug Testing and Development: Engineered tissues can serve as a platform for testing pharmaceutical compounds, allowing for better product development while reducing reliance on animal testing.
These objectives underscore the transformative impact tissue engineering can have on healthcare
Multidisciplinary Nature
The multidisciplinary nature of tissue engineering makes it a unique and powerful field. It draws from various disciplines, including:
- Biology: Understanding cell behavior, tissue architecture, and the body’s healing processes.
- Materials Science: Focusing on the design and application of materials that can serve as scaffolds and support tissue growth.
- Bioengineering: Merging engineering principles to devise solutions that facilitate tissue formation and repair.
- Medicine: Collaborating with medical professionals ensures that engineered tissues meet clinical needs and patient safety regulations.
This integration of knowledge not only enhances innovation but also accelerates the translational process, taking ideas from the laboratory to practical applications in patient treatment.
"The future of tissue engineering is built on the collaborative efforts of diverse fields, driving advancements that can redefine healthcare."
"The future of tissue engineering is built on the collaborative efforts of diverse fields, driving advancements that can redefine healthcare."
The synergy between these disciplines highlights why understanding tissue engineering is pivotal for any stakeholder in biomedicine.
Materials in Tissue Engineering
The materials used in tissue engineering are at the heart of developing functional biological tissues. These materials not only provide a structural framework but also affect how cells grow and function within engineered tissues. Thus, understanding the different types of materials and their properties is crucial for successful tissue regeneration and repair.
Natural Polymers
Natural polymers have emerged as essential components in tissue engineering for several reasons. These materials, derived from biological sources such as collagen, chitosan, and alginate, exhibit biocompatibility, biodegradability, and inherent biological activity. Because they are biologically derived, they often integrate better with host tissues, leading to improved healing outcomes.
- Advantages of Natural Polymers:
- Biocompatibility: Natural polymers are more readily accepted by the body's immune system.
- Biodegradability: They degrade in enzymatic environments, which is often ideal for tissue replacement applications.
- Mimicking Natural Tissues: Their structural properties can resemble the extracellular matrix, promoting better cell adhesion and growth.
However, there are challenges too. Natural polymers can have batch-to-batch variability due to their biological sources, which may compromise the consistency needed for clinical applications. Moreover, their mechanical properties can be less robust compared to synthetic alternatives, which may limit their use in load-bearing applications.
Synthetic Polymers
Synthetic polymers provide a contrasting set of benefits in tissue engineering. Materials like polylactic acid (PLA) and polycaprolactone (PCL) offer tunable mechanical properties and structural integrity, enabling their use in various applications, including scaffolds for bone and cartilage engineering. The advantages of synthetic polymers include:
- Controlled Properties:
- Mechanical Strength: By adjusting the formulation, synthetic polymers can be designed for high-stress applications.
- Longevity: They can be engineered for slower degradation rates, making them suitable for long-term applications.
Despite these benefits, they often lack the biological functionality found in natural polymers. This could hinder cellular interaction and integration with body tissues. Additionally, the potential for inflammation and foreign body reactions poses risks in using synthetic materials for tissue engineering.
Ceramic-based Scaffolds
Ceramic-based scaffolds, such as hydroxyapatite and bioglass, have gained traction due to their favorable properties that encourage bone regeneration. These materials are often bioactive, promoting a chemical bond with bone, which is vital for orthopedic and dental applications.
Some key characteristics of ceramic-based scaffolds are:
- Osteoconductivity: Facilitating the growth of new bone tissue.
- Mechanical Strength: Many ceramics can withstand significant loads, making them ideal for bone applications.
However, living tissues require some flexibility, and ceramics are typically brittle. This may limit their standalone use in dynamic environments where mechanical properties need to be optimized to encourage both durability and flexibility.
"Choosing the right materials is not merely a technical decision; rather, it involves understanding the interplay between biological requirements and material properties."
"Choosing the right materials is not merely a technical decision; rather, it involves understanding the interplay between biological requirements and material properties."
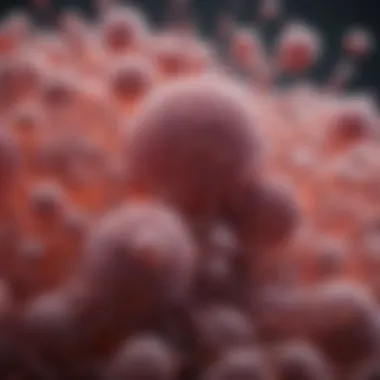
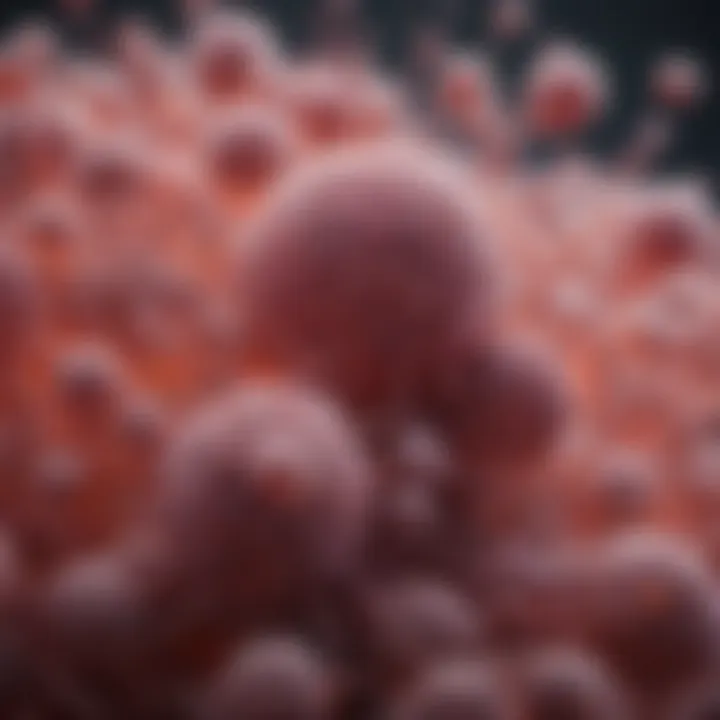
Cellular Components in Tissue Engineering
The cellular components in tissue engineering play an essential role in the development of functional biological tissues. They are not just mere players on the field; they are the very foundation on which the whole game is built. Understanding these components—particularly stem cells, differentiated cells, and their sources—enables researchers and practitioners to harness cellular potential for innovative solutions in regenerative medicine.
Stem Cells
Stem cells are often hailed as the backbone of tissue engineering. Their unique ability to self-renew and differentiate into various cell types makes them invaluable for creating tissues. You could think of them as the versatile actors in a play, ready to take on different roles as the script requires. These cells can evolve into muscle, nerve, or even blood cells, depending on the microenvironment they find themselves in.
The potential benefits of utilizing stem cells in tissue engineering are plentiful:
- Regenerative Capabilities: Stem cells can regenerate damaged tissues, which is particularly crucial in fields like orthopedics and cardiology.
- Disease Model Creation: They help create models for diseases, allowing for better understanding and treatment strategies.
- Personalized Medicine: Patients can receive personalized grafts derived from their own stem cells, minimizing rejection risks.
However, there are considerations surrounding their use. Ethical concerns about the sourcing of embryonic stem cells, regulatory challenges, and the need for strict quality control are just a few hurdles that persist.
Differentiated Cells
Differentiated cells, on the other hand, are specialized cells that have already committed to a specific lineage. While stem cells bring flexibility and potential, differentiated cells are akin to seasoned players who know their positions well. They can be muscle cells, skin cells, or neurons—all critical in rebuilding functional tissues.
Using differentiated cells carries some distinct advantages:
- Immediate Functionality: As they are already specialized, these cells can immediately contribute to the intended function without requiring further maturation.
- Reduced Risk of Tumor Formation: Unlike stem cells, differentiated cells have a lower risk of uncontrolled proliferation, which is a critical factor when considering the safety of implants.
However, the challenge with using differentiated cells lies in their sourcing and the complexity involved in isolating them without losing their specialized characteristics. Ensuring that these cells can maintain their functionality over time is also a key concern.
Cell Sources and Isolation
Identifying and isolating the right cells is a pivotal step in any tissue engineering project. There are various sources of cells, including:
- Autologous Sources: Cells harvested from the patient, such as skin or fat tissue, which reduce the risk of rejection.
- Allogeneic Sources: Cells derived from donors, which can be beneficial when time is of the essence.
- Xenogeneic Sources: Cells from different species, though this approach raises significant ethical and immunogenic concerns.
Isolation techniques can range from traditional enzymatic methods to more advanced mechanical processing. Each method brings its own handful of pros and cons, often influenced by the desired cell type and application.
"The quality and type of cells selected for culture are paramount, as they dictate the functionality and viability of the engineered tissues."
"The quality and type of cells selected for culture are paramount, as they dictate the functionality and viability of the engineered tissues."
The landscape of cellular components in tissue engineering is both promising and complex. By carefully considering the choice of stem cells, differentiated cells, and sourcing methods, researchers can pave the way for groundbreaking applications in regenerative medicine and beyond.
Bioreactors and Culture Systems
In the realm of tissue engineering, bioreactors and culture systems play a crucial role by providing the necessary environment for cells to thrive and produce tissues mimicking natural biological structures. These systems simulate physiological conditions, allowing researchers and engineers to manipulate various factors that influence cell behavior, growth, and differentiation. The importance of bioreactors cannot be overstated, as they are central to accelerating the development of engineered tissues suitable for therapeutic applications.
Types of Bioreactors
Bioreactors can be classified into several types based on their design and function. Understanding the nuances between these types provides insight into how they can be optimized for specific tissue engineering applications:
- Stirred-Tank Bioreactors: These are one of the most widely used types, where cells are suspended in a nutrient-rich medium, allowing for good mass transfer and mixing. They are valuable for creating larger volumes of tissue.
- Membrane Bioreactors: This type utilizes a semipermeable membrane to facilitate the exchange of nutrients and waste. It’s often employed in applications that require keeping cells in a specific microenvironment.
- Fixed-Bed Bioreactors: In this system, cells are anchored to a support matrix, creating a stable environment favorable for tissue formation. This design is particularly effective in mimicking natural tissue architecture.
- Perfusion Bioreactors: Here, continuous flow of media through the scaffold provides nutrients and oxygen while removing waste products. This type supports a more natural cell growth pattern and is beneficial for complex tissue constructs.
"The choice of bioreactor can influence cellular response and the overall success of tissue engineering efforts, impacting both growth rates and tissue functionality."
"The choice of bioreactor can influence cellular response and the overall success of tissue engineering efforts, impacting both growth rates and tissue functionality."
Dynamic vs. Static Culturing
Culturing methods in bioreactors greatly affect cell behavior and tissue development. From this perspective, it is vital to distinguish between dynamic and static culturing methods.
- Dynamic Culturing: This approach involves the application of external forces, such as fluid flow or mechanical stimulation, to promote cell activity and mimic in vivo conditions better. For instance, dynamic culture systems can enhance nutrient uptake and waste removal, ultimately leading to improved cellular function and tissue quality.
- Static Culturing: Conversely, static methods allow cells to grow without any flow or agitation. While simpler, static systems may not adequately reproduce the conditions found in living organisms, which could affect tissue maturation. It is often used in preliminary stages of research or when specific cell types are being studied without the influence of mechanical forces.
Determining the best approach—dynamic or static—depends on the specific objectives of the tissue engineering project and the desired properties of the engineered tissue. Each has its advantages and limitations that can substantially influence the success of tissue constructs.
Engineering Strategies
When it comes to tissue engineering, engineering strategies play a pivotal role in bridging the gap between understanding biological systems and designing functional tissues. These strategies guide researchers and practitioners in developing methodologies that align with the complex structure and function of the tissues they seek to replicate or enhance.
Utilizing these strategies not only helps in effective tissue design but also allows for customization based on specific patient needs, leading to personalized medicine. The ability to adapt strategies to meet individual requirements can vastly improve outcomes in regenerative therapies. Moreover, approaches must be carefully considered in their selection, as they often dictate the success of various projects.
"Choosing the right engineering strategy is like selecting the best tool for a job; it can make or break the outcome."
"Choosing the right engineering strategy is like selecting the best tool for a job; it can make or break the outcome."
Top-Down Approaches
Top-down methodologies generally begin with larger structures or systems. They work by deconstructing these systems into finer components that can then be analyzed and manipulated. This method can be especially beneficial in mimicking how natural tissues are organized. Often referred to as a macro-to-micro strategy, it allows researchers to understand global dynamics before delving into specific cellular functions.
Benefits of Top-Down Approaches include:
- Comprehensive Insight: Engaging with the larger system provides a contextual framework, making it easier to understand spatial arrangements.
- Scalability: These processes can often be scaled up or down with relative ease, thus catering to a range of applications.
- Holistic Development: It ensures that tissue development considers external influences, such as biomechanical and biochemical factors.
However, top-down strategies may not always offer insights into the cellular mechanisms at play, potentially overlooking unique characteristics of smaller scale interactions.
Bottom-Up Approaches
In contrast, bottom-up techniques focus on assembling tissues from the smallest components, like cells and molecules. This strategy emphasizes the importance of cellular behavior and how it contributes to the larger tissue structure. By starting small, researchers can study interactions at the micro-level, allowing for a profound understanding of how cells communicate and function together.
Key Aspects of Bottom-Up Approaches are:
- Precision Engineering: Enables control over cellular environment, which helps in fine-tuning responses.
- In-depth Analysis: Understanding cellular mechanisms offers valuable insights into tissue behavior.
- Innovation Potential: Bottom-up methods often lead to groundbreaking developments as scientists can explore novel combinations of materials and technologies.
However, this approach can also face challenges, such as the complexities involved in cell sourcing and the sometimes unpredictable nature of biological responses.
Through a thoughtful application of both top-down and bottom-up strategies, biotechnology and tissue engineering can develop robust systems capable of addressing a variety of challenges faced in regenerative medicine and beyond.
Applications of Tissue Engineering
Tissue engineering, at its core, is about more than just creating new tissues; it embodies the promise of restoring lost functions and improving human health. A wide array of applications stem from this technological marvel, each bringing unique contributions to medical practices and patient outcomes. Understanding these applications is pivotal in grasping how tissue engineering is not merely a theoretical concept but a practical solution to real-world health challenges.
Regenerative Medicine
Regenerative medicine stands as one of the most significant applications of tissue engineering. This field aims to repair, replace, or regenerate damaged tissues and organs using advanced techniques and biological materials. By drawing on the principles of tissue engineering, regenerative medicine allows for the potential repair of conditions that were once seen as untreatable.
This approach holds promise for varied conditions, such as:
- Cardiovascular diseases: By regenerating heart tissues following ischemic injuries.
- Orthopedic applications: Reconstruction of cartilage and bone tissues to mend injuries or deformities.
- Skin regeneration: Offering solutions for burn victims or chronic wounds, with skin grafting potentially using bioengineered skin.
"Regenerative medicine bridges the gap between biological science and patient care, providing innovative solutions for debilitating conditions."
"Regenerative medicine bridges the gap between biological science and patient care, providing innovative solutions for debilitating conditions."
The major advantage here is the capability to utilize a patient’s own cells, minimizing the risk of rejection that often comes with transplants. This autologous approach is crucial, especially in personalized medicine, leading to better integration of the engineered tissues into the patient’s body.
Drug Testing and Development
Another prominent application of tissue engineering unfolds within drug testing and development. Traditional methods of testing pharmaceutical compounds have profound limitations, often relying on animal models or cell lines that may not accurately predict human responses. Here, tissue-engineered platforms emerge as a revolutionary tool, enabling the construction of more representative models of human tissues.
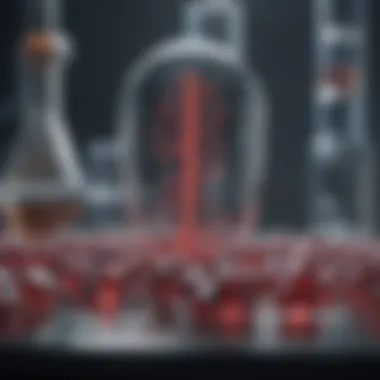

Advantages of this approach include:
- Predictability: More accurate results in assessing drug efficacy and toxicity.
- Efficiency: Reduced time frames in drug development processes by yielding faster and more reliable outcomes.
- Ethical considerations: Alleviating some ethical dilemmas associated with animal testing.
In designing such models, researchers can replicate the complexity of human organs, making it easier to understand how drugs interact at a cellular level. This leap could help in the earlier identification of adverse reactions, ultimately leading to safer therapeutic agents reaching the market.
Organ Transplantation
The concept of organ transplantation often evokes a range of emotions, tied closely to the scarcity of available organs and the immense waiting lists that exist. Tissue engineering offers a glimmer of hope in addressing this critical issue by providing a pathway to developing bioengineered organs. This application has transformative potential, not just in increasing the quantity of available organs, but also ensuring higher compatibility and functionality.
Key aspects related to this application include:
- Bioengineered organs: Such as kidneys, livers, and even hearts produced from stem cells or other cellular sources, potentially negating the need for donors.
- Customization: Tailoring organs to fit the specific biological makeup of the recipient, reducing the risk of rejection.
- Sustainability: Offering a sustainable alternative that could alleviate the ongoing shortages and tragic consequences of transplant waiting lists.
While the field is still maturing, the strides made so far in organ engineering lay down a foundation that could reshape transplant medicine, saving lives and altering treatment paradigms.
In summary, the applications of tissue engineering are vast and varied. From regenerative medicine to drug testing and organ transplantation, each facet carries its unique set of advantages and considerations. As the technologies evolve, so too does the potential for these applications to drive meaningful changes in healthcare, presenting innovative solutions to challenges that have long plagued the medical community.
This journey into tissue engineering not only highlights its importance but also underscores the critical need for ongoing research, investment, and ethical deliberation, ensuring that such advancements serve their intended purpose: improving the quality of life.
Ethical Considerations in Biotechnology
The landscape of biotechnology is not only shaped by its advancements but also by the ethical considerations that accompany these developments. As biotechnology, particularly tissue engineering, evolves, it prompts a re-examination of moral and ethical boundaries. These considerations are crucial for fostering trust and integrity within the scientific community and beyond.
Life sciences often engage with complex questions that stir public sentiment. Stakeholders, including researchers, ethicists, and policymakers, must navigate a labyrinth of dilemmas. Consequently, ethical considerations become a cornerstone in discussions about advancements in biotechnology, guiding responsible practices and ensuring the welfare of society.
"Ethics is knowing the difference between what you have a right to do and what is right to do."
"Ethics is knowing the difference between what you have a right to do and what is right to do."
Ethical Dilemmas
Among the many ethical dilemmas faced in biotechnology, questions regarding genetic manipulation stand tall. Should scientists have the capability to alter the genetic makeup of organisms, including humans? This question presents a dual-edged sword. On one side, advancements like CRISPR technology promise breakthroughs in treating genetic disorders. On the other, the potential for misuse looms large—designer babies and unintended consequences present real risks.
- Informed Consent: Another pressing concern arises with informed consent, especially when dealing with stem cell research. Individuals donating cells or tissues must thoroughly understand potential outcomes, risks, and benefits.
- Animal Welfare: The use of animals for testing in biotechnological research raises further ethical considerations. The harm inflicted on research subjects must be minimized, aligning with a broader ethical obligation to ensure humane treatment.
- Environmental Impact: As biotechnology pushes into new frontiers, the ramifications on biodiversity and ecosystems are significant. Unintended consequences, such as genetically modified organisms affecting native species, must be scrutinized before widespread application.
Regulatory Framework
Establishing a robust regulatory framework is imperative in addressing the ethical challenges posed by biotechnology. Regulatory bodies serve as custodians, ensuring that biotechnological advancements occur within ethical boundaries, fostering trust in scientific exploration.
In various countries, regulations governing biotechnology differ significantly, shaped by cultural, ethical, and social mores. For instance:
- USA: The Food and Drug Administration (FDA) and the Environmental Protection Agency (EPA) oversee genetic engineering in food products and drugs, enforcing safety and efficacy standards.
- EU: Tighter regulations exist regarding genetically modified organisms (GMOs), focusing heavily on precautionary principles and public health considerations.
- International Bodies: Organizations like the World Health Organization (WHO) and UNESCO set guidelines that transcend national boundaries, aiming for global ethical standards in biotechnological research.
These regulatory structures help mitigate risks by requiring comprehensive risk assessments and promoting transparency in research. They also establish ethical protocols for experimentation and the dissemination of biotechnology products, reflecting the collective moral stance of society.
Moving forward, a collaborative effort across borders and disciplines is necessary to adapt regulations to the rapidly changing biotechnological landscape. Only then can we harness the benefits of advancements responsibly, ensuring that societal values and ethical considerations remain at the forefront of biotechnological innovation.
Future Directions in Tissue Engineering
The landscape of tissue engineering is ever-changing, influenced by advancements in technology and scientific understanding. This section looks at the future of the field, focusing on innovative technologies and how they will integrate with genomics and proteomics. These advancements hold the potential to revolutionize the current practices, making them more precise, efficient, and accessible for clinical applications.
Innovative Technologies
The future of tissue engineering hinges on a blend of innovation and traditional techniques. Emerging technologies such as 3D bioprinting are set to be game-changers. 3D bioprinting combines the concepts of tissue engineering with printing technologies, allowing for the layer-by-layer fabrication of tissue structures. Here are some noteworthy elements of this innovation:
- Precision: The ability to create complex structures that closely mimic natural tissues. This accuracy enhances the functionality of engineered tissues.
- Customization: Personalized medicine is on the horizon. Tailored tissues that match a patient’s biological makeup can significantly improve transplant success rates.
- Speed: Rapid prototyping of tissues reduces the time from development to clinical application. This could mean quicker solutions to pressing health issues.
Another promising avenue is the use of organ-on-chip technologies. These miniature devices simulate the functions of whole organs, providing valuable platforms for drug testing and disease modeling. They help in:
- Reducing reliance on animal models, thus addressing some ethical concerns surrounding animal testing.
- Improving predictive accuracy for how a drug will behave in a human body, enhancing drug development processes.
"These technologies are not just about creating tissues but also understanding the complexities of human biology on a granular level."
"These technologies are not just about creating tissues but also understanding the complexities of human biology on a granular level."
In addition, advancements in CRISPR and gene editing offer immense possibilities. By enabling precise modifications to the DNA of stem cells, we can engineer specific cell behaviors and functionalities. This can lead to
- Enhanced regenerative capabilities
- Improved integration of transplanted tissues or organs
These innovative technologies collectively signal a new era where the line between technology and biology is more integrated than ever before.
Integration with Genomics and Proteomics
As biotechnology progresses, the crossroad of tissue engineering with genomics and proteomics becomes increasingly evident. This integration is crucial for understanding cellular functions and responses, which is paramount in the engineering of tissues that closely mimic natural ones.
Genomics—the study of an organism's complete set of DNA—provides insights into genetic variations that can affect tissue development. By understanding these genetic blueprints, scientists can:
- Identify specific genes responsible for tissue growth and repair, enabling targeted therapies that address specific medical conditions.
- Use genomic data to inform the design of biomaterials that can elicit desired biological responses, thus enhancing the efficiency of tissue engineering strategies.
On the other hand, proteomics, which examines the complete set of proteins produced by an organism, offers insights into how cells respond to their environment. Integrating proteomic analyses into tissue engineering can lead to:
- Better understanding of cell signaling pathways, which is essential for guiding the differentiation of stem cells into specific cell types.
- Enhanced ability to tailor scaffolds in ways that promote specific protein expressions crucial for tissue functionality.
Case Studies in Biotechnology
Understanding the practical applications of biotechnology is greatly enhanced through case studies. These real-world examples allow students, researchers, educators, and professionals to connect theory with practice. They illustrate the successes and challenges faced within this evolving field, offering valuable insights into methodologies, ethical considerations, and innovative technologies. By examining specific instances of tissue engineering projects, one can evaluate the effectiveness of various approaches and materials used in bioengineering.
Case studies serve as a bridge linking scientific concepts with tangible outcomes. This not only reinforces learning but also inspires future innovations. They demonstrate how biotechnology can solve complex medical issues or improve human health, making the discipline more relatable and engaging for a wider audience.
Successful Tissue Engineering Projects
Successful tissue engineering projects exemplify the potential of biotechnology in medicine. One stand-out example is the development of skin substitutes for burn victims. Graftskin is a notable product made from living skin cells, which has been successfully used to treat burn injuries and chronic wounds. This graft helps in regenerating tissue and promotes healing significantly faster than traditional dressings, providing patients with a viable alternative.
Another remarkable project is the engineering of bladders from patients' own cells using a technique known as autologous bladder augmentation. Researchers at the University of Wake Forest have demonstrated that, by combining stem cells with biocompatible scaffolds, they could create functional tissue that mimics natural bladder properties. This not only shows the elegance of using a patient’s own cells but also highlights the reduced risk of rejection by the immune system.
Other successful instances can be seen in the realm of cartilage regeneration. The implementation of a hyaluronic acid-based scaffold in conjunction with stem cells has led to notable successes in repairing damaged cartilage in knees, enabling patients to regain mobility.
Lessons Learned from Past Research
Reflecting on past research in biotechnology reveals vital lessons that can shape future endeavors. One critical takeaway pertains to the importance of thorough preclinical studies. Many promising projects have stalled or failed when translated from laboratory settings to clinical applications. A glaring example is the initial excitement around embryonic stem cell therapies. While they exhibited potential for regenerative medicine, many projects faced considerable regulatory and ethical hurdles that hindered their progress.
Moreover, trials like the use of myoblasts to regenerate heart tissue have taught researchers the significance of understanding the microenvironment. The failure of these trials has emphasized the need for scaffolds that not only support cell growth but also mimic the natural cellular milieu more accurately.
"Past failures are oftentimes the best teachers of success. Every setback reminds scientists of the unpredictable nature of biological systems and the need for adaptability in research."
"Past failures are oftentimes the best teachers of success. Every setback reminds scientists of the unpredictable nature of biological systems and the need for adaptability in research."
The journey through biotechnology is punctuated by both triumphs and setbacks. Learning from these instances will pave the way for improved methodologies, ultimately refining the art and science of tissue engineering. As we proceed, the blending of data-driven approaches alongside hands-on laboratory techniques can lead to groundbreaking advancements in how we understand and apply biotechnology in real-world scenarios.
Challenges in Biotechnology and Tissue Engineering

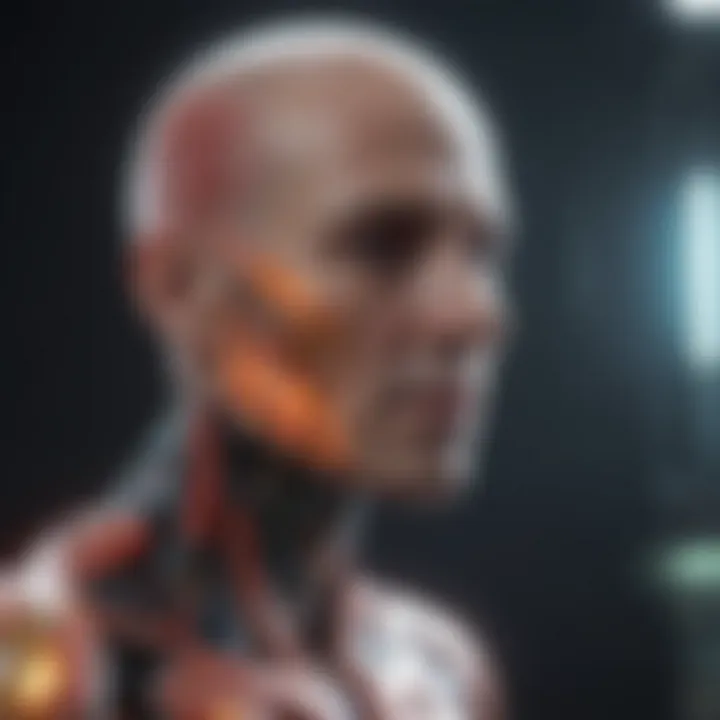
Biotechnology and tissue engineering hold breathtaking promise. However, complexities also come hand in hand with potential. It’s vital to acknowledge the substantial challenges that accompany these fields, as they can dictate both the pace of advancements and the overall success in therapeutic applications. Examining these issues thoroughly is essential, as they influence not only technological innovation but also societal acceptance and regulatory compliance.
Technical Challenges
In the realm of tissue engineering, the technical hurdles are conspicuous and complex. One major problem is achieving successful integration of engineered tissues with the host environment. Scientists often grapple with issues like vascularization, the process of forming new blood vessels. Without an adequate blood supply, even the most sophisticated tissue designs can fail to survive after implantation.
Moreover, scaffold fabrication is fraught with complications. Creating scaffolds that mimic the intricacies of natural tissues in terms of mechanical properties, porosity, and biodegradability remains a significant roadblock. If these scaffolds aren't tailored to match the physical characteristics of their target tissues, the engineered constructs may not function as intended post-implantation.
Another thorny issue pertains to cell sourcing. Obtaining the right type and quantity of cells for fabricating tissues is often a significant bottleneck. Stem cells might offer a solution, but variability in cell sources and differentiation pathways can create inconsistent outcomes. This inconsistency complicates the predictability and reproducibility that researchers strive for in their studies, making it difficult to scale up processes or to translate findings from the lab to clinic.
"Technical hurdles must be overcome to realize the potential of tissue engineering in medical treatments."
"Technical hurdles must be overcome to realize the potential of tissue engineering in medical treatments."
Regulatory Hurdles
Alongside technical challenges, regulatory complications cast a long shadow across biotechnology and tissue engineering projects. The landscape of regulations is often complex and multifaceted, especially when it comes to approving innovative therapies for human use. Each country has differing guidelines, and navigating these can be a daunting task for researchers and companies alike.
One significant aspect is safety and efficacy evaluation. Before a new tissue-engineered product can hit the market, it must endure rigorous testing to assure its safety for patient use. Regulatory frameworks like the U.S. Food and Drug Administration (FDA) require extensive documentation and testing, which can drag projects through lengthy approval processes. This impacts timelines, budgets, and even the feasibility of certain innovative ideas.
Additionally, there is often a lack of clear guidance from regulatory bodies on how to classify and assess tissue-engineered products. Without standardized protocols, developers may find themselves in limbo, unable to move forward because the rules of the game aren’t well defined.
Lastly, public perception and ethical debates around biotechnology can complicate regulatory processes. Public concerns over the potential misuse of biotechnological advancements often lead to stricter scrutiny, forcing regulatory bodies to tread carefully. Balancing innovation with public trust and safety becomes a tightrope walk that stakeholders must navigate continually.
Ecosystem of Biotechnology
The ecosystem surrounding biotechnology plays a pivotal role in the advancements in this remarkable field, specifically concerning tissue engineering. This ecosystem comprises not only the scientific community but also industries, regulatory agencies, and educational institutions that interlink to foster innovation and development. Each component of the ecosystem contributes uniquely, creating a synergistic environment that drives research and practical applications forward.
Industry Players
The landscape of biotechnology is rich with various industry players who bring diverse skills and resources to the table. These players can predominantly be categorized into several types:
- Biotechnology Firms: Companies like Amgen and Genentech lead in developing biotechnological products, including therapeutics that increasingly integrate with tissue engineering techniques. Their role in pushing technological boundaries cannot be overstated.
- Pharmaceutical Companies: Major players such as Pfizer and Novartis are investing in biotechnology to enhance drug development processes. They often collaborate with biotech firms to optimize drug delivery through engineered tissues.
- Academic and Research Institutions: Universities such as MIT or Stanford are hotbeds of innovation, cultivating talent and conducting research that lays the groundwork for breakthroughs in biotechnology. These institutions often partner with industry to translate research into viable products.
- Government Agencies: Agencies like the National Institutes of Health (NIH) and the Food and Drug Administration (FDA) provide essential support through funding and regulatory oversight, ensuring that new biotechnological advancements meet safety standards.
Together, these entities not only compete but also collaborate to enhance the field's direction and applicability. In doing so, they develop new methods and applications that might have previously been considered mere science fiction.
Collaborative Networks
In biotechnology, collaborative networks are crucial for fostering innovation and addressing complex challenges that no single entity can solve alone. These networks enable sharing of knowledge, resources, and technology among various stakeholders. Several essential points about these networks include:
- Interdisciplinary Collaboration: The intersection of various fields, such as bioinformatics, materials science, and engineering, enhances the development of more efficient tissue engineering techniques. Scientists often work alongside engineers and medical professionals to bring ideas to fruition.
- Public-Private Partnerships: Collaborations between public institutions and private companies often expedite research and development processes. For example, the partnership between the FDA and various biotech companies has streamlined procedures for getting novel therapeutics to market.
- Global Reach: With the prevalence of digital communication tools, these networks are not confined to local or national levels. They have a broad global outreach that encourages diverse cultural perspectives, which can lead to more holistic solutions to biotechnological issues.
"The strength of biotechnology lies not only in individual players but in the collaborative efforts that propel the field forward."
"The strength of biotechnology lies not only in individual players but in the collaborative efforts that propel the field forward."
Collaborative networks shorten the time needed for concept-to-market transitions while maximizing the strengths of each entity involved. As a result, these networks are vital in ensuring that biotechnology not only thrives as an industry but also remains at the cutting edge of scientific discovery and application.
Educational Aspects of Biotechnology
The world of biotechnology is like a vast ocean, brimming with life, innovations, and possibilities. Educational aspects of this field hold a crucial role, as they lay the groundwork for the next generation of professionals who will navigate, explore, and expand this domain. By integrating theoretical concepts with practical applications, education in biotechnology fosters an environment where students can learn about the intricate mechanics of living systems and their manipulations.
Academic Programs
In recent years, educational institutions have established a variety of academic programs tailored to the booming field of biotechnology. These range from undergraduate degrees to specialized master’s and even doctorate programs.
Key features of academic programs include:
- Interdisciplinary Approach: Programs often blend biology with engineering, chemistry, and data science. This helps students understand the multifaceted nature of biotechnology.
- Hands-on Training: Many programs emphasize laboratory work, providing students with an opportunity to engage directly with techniques such as DNA extraction and tissue culturing.
- Industry Collaboration: Partnerships with biotech companies can enhance educational programs, allowing students to work on real-world projects and internships.
Institutions also offer certifications in niche areas such as bioinformatics and tissue engineering, which can be beneficial in keeping up with industry demands. As a result, graduates emerge well-prepared to address the challenges of modern biotechnological applications.
Research Opportunities for Students
The research landscape in biotechnology is constantly evolving, and it presents a myriad of opportunities for students. Participating in research enhances theoretical knowledge and hones essential skills that are invaluable in the workforce.
Considerations for engaging in research include:
- Mentorship: Having mentors in research labs can provide students with guidance and insight. Such relationships are key in developing investigation skills and critical thinking.
- Interdisciplinary Projects: Collaborative research often brings together students from various backgrounds. This opens up avenues for innovative approaches and comprehensive problem-solving.
- Publication Opportunities: Students involved in research may eventually publish their findings. This involvement not only looks great on a resume but also contributes to the global conversation in biotechnology.
"Education is the passport to the future, for tomorrow belongs to those who prepare for it today." - Malcolm X
"Education is the passport to the future, for tomorrow belongs to those who prepare for it today." - Malcolm X
Through a blend of theoretical knowledge and practical experience, the educational framework in biotechnology ensures that graduates are ready to tackle real-world challenges and push boundaries in this transformative field.
Public Perception of Biotechnology
Public perception of biotechnology plays a pivotal role in the progress and acceptance of innovations in this realm, particularly in tissue engineering. Understanding how individuals and communities view these biotechnological advancements affects everything from funding opportunities to the implementation of new therapies. Societal attitudes shape regulations, influence market dynamics, and ultimately determine the trajectory of research and development. Many people are cautious about biotechnological applications, stemming from ethical concerns, misunderstandings, and fear of unforeseen consequences. Thus, an effort to bridge the gap between scientific truth and public sentiment is increasingly essential.
Cultural Context
Cultural factors significantly influence how biotechnology is perceived. In some societies, biotechnology is embraced wholeheartedly, seen as a means to improve health outcomes, enhance food security, and tackle pressing environmental challenges. People might relate these advancements to their cultural narratives regarding health and wellness. For instance, communities with a strong tradition of herbal medicine may be more open to biotechnological solutions that integrate natural elements, while others may resist technologies perceived as unnatural or overly invasive.
In other regions, suspicions around genetic modification or stem cell research have deep historical roots, often tied to religious or philosophical beliefs. These sociocultural frameworks contribute to a polarized perception where biotechnology is celebrated in some circles while vilified in others. Research conducted by organizations like the Pew Research Center indicates that cultural attitudes can shift dramatically based on how information is presented in the media or by public figures, showcasing the fluid nature of public opinion.
Communication Strategies
Effective communication strategies are crucial for fostering a positive public perception of biotechnology. Engaging the public in dialogue about biotechnological developments through transparent communication is vital.
- Educational Outreach: Initiatives that provide clear, accessible information about biotechnology can demystify the subject. Workshops, community discussions, and social media platforms serve as effective avenues for education.
- Inclusive Dialogue: Involving stakeholders from diverse communities ensures that communication addresses specific concerns and motivations, thereby enhancing resonance with target audiences. By embracing local dialects and cultural frameworks in messages, scientists can better connect with the public.
- Leveraging Social Media: Platforms like Facebook and Reddit can amplify positive narratives surrounding biotechnology. They serve as interactive spaces where individuals can ask questions and share experiences, reducing misinformation and increasing trust.
Overall, fostering a constructive public perception of biotechnology is an ongoing challenge that requires sensitivity, adaptation, and a willingness to engage with diverse audiences. Exploring the cultural context and implementing targeted communication strategies can significantly enhance public understanding and acceptance of the biotechnological advancements that hold the potential to revolutionize both science and health.
Integration of Artificial Intelligence in Tissue Engineering
The incorporation of artificial intelligence (AI) into tissue engineering marks a significant turning point in the field. By combining biological expertise with computational power, AI offers a suite of tools that can optimize various processes within tissue engineering. This integration is not merely a trend; it’s a fundamental shift that could shape the future of regenerative medicine, bringing with it a range of specific elements, noteworthy benefits, and critical considerations.
One of the prominent areas where AI is making waves is in predictive modeling. This is where machine learning algorithms come into play, analyzing vast datasets to predict how engineered tissues will behave in real-world scenarios. Such modeling allows researchers to simulate different conditions and the resultant tissue responses without the need for extensive physical experiments. This can save time, resources, and even minimize the ethical concerns associated with animal testing, providing insights much quicker than traditional methods. Imagine the efficiency of predicting outcomes and improving design iterations before moving onto the costly and time-consuming phases of actual experiments.
Predictive Modeling
Predictive modeling harnesses the power of AI to foresee how tissues will react under varying conditions. For instance, when creating a new skin graft, a predictive model can account for factors like moisture retention, elasticity, and even wound healing efficiency. The benefits here are multifaceted:
- Reduced Experimentation: Bringing down the number of trial-and-error experiments saves both costs and time.
- High Precision: AI algorithms can analyze complicated data sets far beyond human capacity, leading to more accurate predictions.
- Customized Outcomes: The ability to tailor tissue properties based on patient-specific data enhances the personalization of medical treatments.
AI's capacity to uncover patterns and relationships within biological data enhances the overall trajectory of tissue engineering projects. As researchers train AI systems on historical data, these models improve over time, refining their predictions and helping professionals in making informed decisions.
"The future of tissue engineering lies not just in biological understanding, but in harnessing computational power to navigate complex biological systems efficiently."
"The future of tissue engineering lies not just in biological understanding, but in harnessing computational power to navigate complex biological systems efficiently."
Data Analysis in Research
The impact of AI isn’t limited just to predictive modeling; it also extends to the realm of data analysis in research. In tissue engineering, hefty amounts of data are generated from cell cultures, imaging studies, and genetic sequencing. AI enables researchers to analyze this information swiftly, making the process of extracting meaningful insights more manageable.
For example, AI tools can identify trends in cell behavior over time, revealing critical information about how various scaffolding materials affect cellular growth and differentiation. Some notable aspects of AI-enhanced data analysis include:
- Streamlined Data Processing: AI can automate the parsing of large data sets, allowing researchers to focus on interpretation rather than raw number-crunching.
- Enhanced Visualization: Advanced algorithms generate visual patterns that might be missed in traditional datasets, offering clearer hypotheses for future studies.
- Real-Time Insights: The capability to analyze data as it is collected helps in accelerating research timelines and enables immediate course corrections.