Designing Effective PCR Protocols for Various Applications
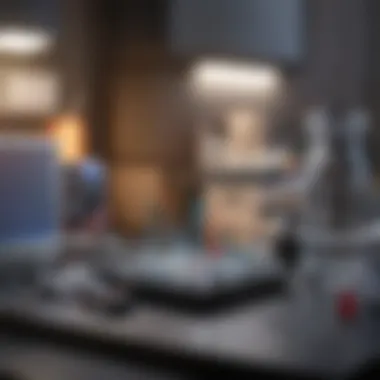
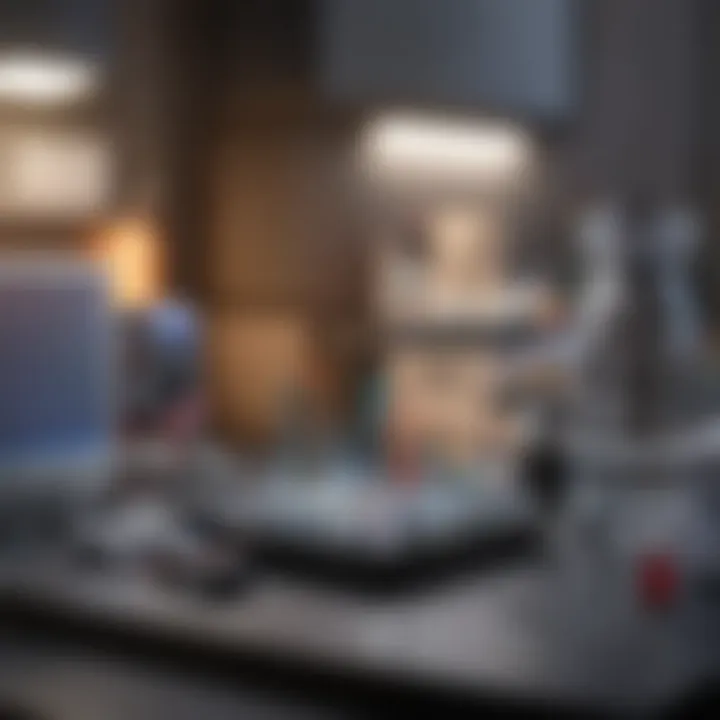
Article Overview
Purpose of the Article
This article aims to unravel the complexity of designing effective polymerase chain reaction (PCR) protocols. As researchers, educators, and students navigate the intricate landscape of molecular biology, a solid grasp of PCR design principles is crucial. By outlining the components required for successful PCR and offering insights into primer design and optimization strategies, this guide sets the groundwork for crafting tailored protocols suited to diverse scientific efforts.
Relevance to Multiple Disciplines
PCR is not just a buzzword; it has become a cornerstone in various fields, including genetics, forensics, and clinical diagnostics. Whether one is amplifying DNA for a genetic study, isolating viral RNA in a medical context, or resolving a forensic examination, the precision of the protocol chosen can have profound implications. Understanding PCR design is therefore indispensable for professionals across multiple disciplines.
Research Background
Historical Context
Since its inception in the 1980s by Kary Mullis, PCR has seen an unstoppable proliferation in its applications. Initially a lab marvel, PCR has evolved into a routine procedure across various settings, from research labs to hospitals. The journey of PCR from a fanciful idea to a pivotal tool in modern science is representative of the ingenuity of scientific progress. It turned molecular biology protocols on their heads and enabled discoveries that have shaped our understanding of genetics and biology.
Key Concepts and Definitions
At its core, PCR is a method used to amplify a specific segment of DNA, allowing scientists to produce millions of copies of a particular gene or sequence. Here are some key terms and concepts that lay the foundation for understanding PCR:
- Template DNA: The DNA that contains the region of interest which you want to amplify.
- Primers: Short sequences of nucleotides that initiate the PCR process by binding to the template DNA.
- DNA Polymerase: The enzyme responsible for synthesizing new strands of DNA.
- Thermal Cycling: The repetitive cycle of heating and cooling that allows denaturation, annealing, and extension of the DNA.
Understanding these concepts provides a groundwork to delve deeper into the intricacies of PCR protocol design. Each component plays an essential role in the final output of the PCR experiment, and their successful integration is what leads to precise, reproducible results.
"The respect for a deep understanding of the components and processes involved in PCR cannot be overstated. It lays the foundation for success in any molecular biology application."
"The respect for a deep understanding of the components and processes involved in PCR cannot be overstated. It lays the foundation for success in any molecular biology application."
The subsequent sections will discuss primer design, optimization strategies, and how all these elements come together to create robust PCR protocols that suit a variety of inquiries.
Foreword to PCR
Polymerase Chain Reaction, or PCR, is an essential technique in molecular biology that enables researchers to amplify small segments of DNA. The significance of PCR in modern science cannot be overstated; it has revolutionized areas such as genetics, forensic science, and medical diagnostics. Understanding the fundamentals of PCR sets the groundwork for developing effective protocols tailored to specific research needs. This section will provide insights into the PCR process and the historical context that shaped its evolution, helping readers appreciate the method's profound implications in scientific inquiry and innovation.
Understanding Polymerase Chain Reaction
PCR is a method that mimics the natural process of DNA replication, allowing scientists to make millions of copies of a specific DNA sequence from just a few input molecules. The procedure primarily involves three key steps: denaturation, annealing, and extension. During denaturation, heat is applied to separate the two strands of DNA. Next comes the annealing phase, where short DNA fragments known as primers bind to the target sequence on each strand. Finally, in the extension step, a specialized enzyme called DNA polymerase synthesizes new strands by adding nucleotides complementary to the target DNA template. This cycle is repeated numerous times, leading to exponential amplification of the targeted DNA segment.
The effectiveness of PCR lies in its precision and ability to amplify minute amounts of DNA, making it indispensable for applications like detecting genetic mutations, cloning genes, and diagnosing infectious diseases. By understanding the mechanics of PCR, researchers can design protocols that enhance yield and specificity, thereby optimizing their experiments.
Historical Context and Development
The journey of PCR began in the early 1980s when Kary Mullis, an American biochemist, first conceptualized the process. Mullis stumbled upon the idea while driving through the Californian countryside, envisioning a way to replicate DNA that would not require cloning in bacteria. He later developed the method and published his findings in a seminal paper in 1985.
From its inception, PCR has undergone significant advancements. Initially, the process was hampered by the instability of polymerases at high temperatures used for denaturation. The breakthrough came with the discovery of Thermus aquaticus, a heat-stable DNA polymerase named Taq polymerase, which could withstand the extreme conditions of PCR. This finding not only simplified the protocol but also boosted its reliability, leading to widespread adoption across various scientific fields.
Today, PCR continues to evolve, with innovations such as real-time PCR, which allows for the quantitative detection of DNA, and multiplex PCR, which can amplify multiple fragments simultaneously. This technological progress exemplifies how PCR has transitioned from a novel laboratory technique to a cornerstone of modern biological research.
"PCR is one of the most significant technological advancements in the field of molecular biology, enabling insights into genetics and diseases that were once considered unattainable."
"PCR is one of the most significant technological advancements in the field of molecular biology, enabling insights into genetics and diseases that were once considered unattainable."
In summary, PCR is not just a method for amplifying DNA; it represents a paradigm shift in molecular biology, fostering advancements in research and diagnostics. Understanding its origins and basic mechanisms sets the stage for exploring the meticulous design and optimization of PCR protocols in subsequent sections.
Fundamental Components of PCR
The Polymerase Chain Reaction, commonly known as PCR, is a vital technique in molecular biology that has revolutionized various fields ranging from clinical diagnostics to forensic science. At its core, PCR is not just about amplification; it hinges on specific components that each play a significant role. Understanding these fundamental components is crucial for designing efficient protocols that lead to accurate and reproducible results. Here, we’ll dive deep into four key elements: the DNA template, primers, DNA polymerase, and the buffer system. Each of these parts works in concert to facilitate the magic of PCR, transforming a tiny fragment of DNA into a sizable quantity that can be analyzed or utilized in several applications.
DNA Template Requirements
The DNA template is the starting material for a PCR reaction. Without it, there would be no target to amplify. It can be extracted from various biological sources, such as blood, tissue, or cell cultures. The quality and quantity of the DNA template are paramount. If the DNA is degraded or contains inhibitors from the extraction process, it can hinder the PCR outcome.
Here are some considerations for selecting a DNA template:
- Concentration: Ideally, template concentrations should fall within the range of 0.1 to 1 ng/µL to achieve optimal amplification.
- Purity: High-quality DNA is critical. A260/A280 ratio should ideally be around 1.8, indicating minimal protein contamination.
- Size: While PCR can amplify fragments of various lengths, the size of the target DNA can affect the efficiency, particularly in terms of extension times.
Investment in quality template preparation sets the stage for a successful PCR and minimizes issues that may arise down the line.
Role of Primers in PCR
Primers are short sequences of nucleotides that bind to specific regions on the DNA template. They are indispensable for initiating the PCR process. By providing a starting point for DNA synthesis, primers define the region of DNA that will be amplified.
Consider the following about primers:
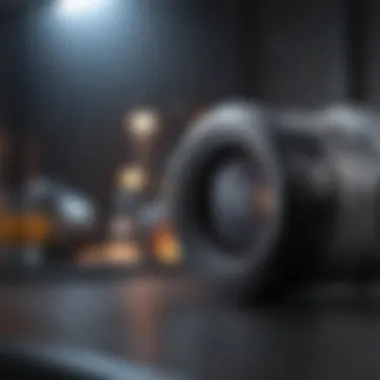
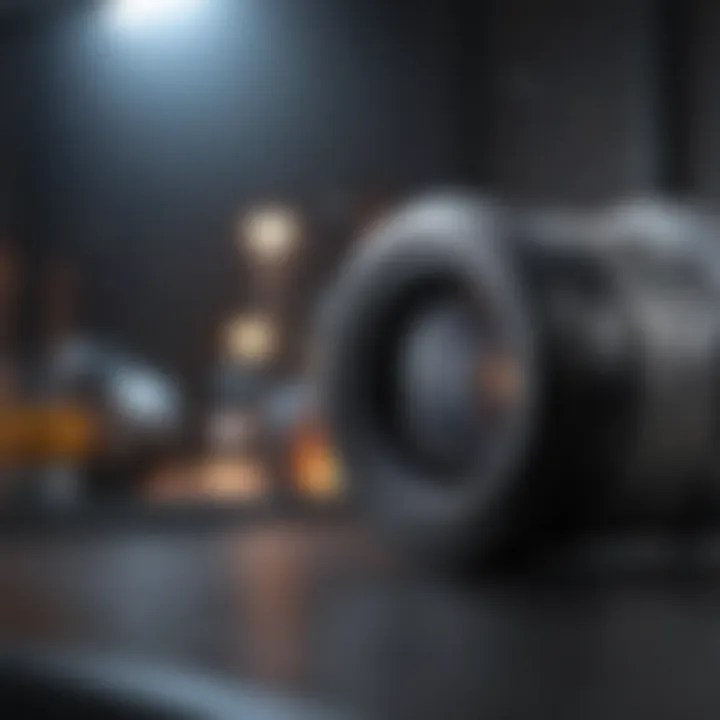
- Design: The specificity of primers is crucial; they should anneal only to the target sequence without forming complexes with each other or non-target DNA.
- Length: Longer primers (typically 18-25 nucleotides) tend to offer greater specificity but can also create issues if they are prone to secondary structures.
- GC Content: An ideal GC content of 40-60% aids in stable binding. Too high or low can lead to melting temperature variations, affecting amplification.
By crafting effective primers, researchers can optimize their PCR results, minimizing non-specific amplifications, and maximizing fidelity.
Importance of DNA Polymerase
DNA polymerase is the enzyme at the heart of PCR, responsible for synthesizing new DNA strands. Selecting the right polymerase is crucial since different polymerases possess unique attributes that can impact the amplification process.
Here are notable characteristics of DNA polymerase:
- Processivity: Some polymerases can synthesize longer DNA strands more rapidly than others. For instance, Taq polymerase is widely used for routine PCR because it is thermostable and works well at high temperatures.
- Fidelity: High-fidelity polymerases like Phusion or Q5 have proofreading abilities, significantly reducing error rates during amplification. This is especially important for applications requiring high accuracy, such as cloning or sequencing.
- Temperature Dependence: Various polymerases function optimally at different temperatures. Knowing the temperature profile of your chosen polymerase can help in setting appropriate cycling conditions.
Understanding the specific capabilities of different DNA polymerases allows for better protocol customizations depending on the intended application.
Buffer and Ion Composition
A well-optimized buffer system is the unsung hero of a successful PCR. The buffer composition aids in maintaining the stability and activity of the enzymes involved, providing an ideal environment for the reaction to occur.
Key components include:
- Tris-HCl Buffer: Typically, Tris is used to maintain the pH, making sure it remains stable throughout the reaction.
- Salt Concentration: NaCl or KCl helps stabilize the DNA double helix structure during annealing. The right salt concentration also enhances primer annealing specificity.
- Divalent Cations: Magnesium ions (Mg2+) are crucial for the activity of most DNA polymerases. The concentration of Mg2+ should be tailored, as too much can cause non-specific amplifications.
- Additives: Sometimes, additives like DMSO or betaine may be employed to improve the yield and specificity, particularly when amplifying GC-rich regions.
Key Considerations in Primer Design
Designing effective primers forms the backbone of successful PCR experiments. Primers are short sequences of nucleotides that initiate the amplification of specific DNA regions. Selecting the right primers ensures not only specificity but also efficiency in the PCR process. A well-designed primer can significantly increase the yield of product and reduce the chance of non-specific amplification, often a major pain point for researchers.
Length and Composition of Primers
When it comes to primer length, striking the right balance is key. Primers typically range from 18 to 25 nucleotides in length. Too short, and they may not bind efficiently, while too long may lead to increased potential for secondary structures. Aiming for a length of around 20 nucleotides is widely regarded as optimal.
- Nucleotide Composition: The GC content should be around 40-60%. This range stabilizes primer binding due to the greater number of hydrogen bonds formed by guanine and cytosine compared to adenine and thymine.
- End Composition: Having a G or C at the 3' end of the primer can provide added stability.
Paying attention to these details can make or break your PCR results.
Melting Temperature (Tm) Calculations
Understanding Melting Temperature or Tm is crucial in primer design. Tm refers to the temperature at which half of the DNA strands are in the double-helix state and half are in the "melted" single-stranded state. Generally, you want the Tm of your primers to be within 2-5 degrees Celsius of each other to ensure that they anneal effectively during the same temperature cycle.
- Calculating Tm: You can calculate Tm using the following formula:
[ Tm = 81.5 + 0.41 \times (G+C) - 675/L ]
where L is the length of the primer in nucleotides. Several online tools can assist with this calculation, making it a lot easier for researchers.
- Buffering Tm: Be mindful that the presence of salts in your buffer can also increase Tm values, so adjustments may be needed.
Avoiding Secondary Structures
Secondary structures in DNA, such as hairpins or dimers, can derail a PCR run completely. These structures hinder the efficiency of amplification, leading to lower yield or even failed reactions. Therefore, it is essential to check for potential secondary structures during the design phase.
- Hairpin Loops: A hairpin loop occurs when the primer binds to itself rather than to the target DNA, thus compromising the reaction.
- Dimer Formation: When two primers bind to each other instead of their target, this can also lead to failed amplification. Tools like OligoAnalyzer can help predict potential secondary structures by analyzing your primer.
Specificity and Inclusiveness
In designing primers, you want them to be specific enough to target the desired sequence while still remaining flexible enough to accommodate variations in that sequence. This balance is crucial.
- Avoiding Homologous Sequences: A primer that binds to multiple targets can create unwanted amplification. Using tools like BLAST can help identify homologous sequences.
- Inclusiveness for Variability: Consider primer sets that can potentially capture a range of allele variants. This is especially useful in research focusing on SNPs or mutations in clinical diagnostics.
By keeping an eye on these aspects of primer design, you lay the groundwork for effective PCR. As such, thoughtful design not only enhances product yield but also ensures that your research pushes the envelope rather than hits a roadblock.
"A primer is not just a sequence; it’s the key that unlocks your experiment’s potential."
"A primer is not just a sequence; it’s the key that unlocks your experiment’s potential."
By focusing on these critical considerations, you can significantly improve the likelihood of success in your PCR protocols.
Optimization of PCR Conditions
The significance of optimizing PCR conditions cannot be overstated. Just as a chef tweaks ingredients to enhance the flavor of a dish, researchers must finely tune PCR settings to achieve the desired outcomes in DNA amplification. Effective optimization results in higher specificity, improved yield, and reliable reproducibility of PCR products, which are essential for any scientific inquiry utilizing this method. In this section, we'll delve into several pivotal components of PCR optimization, highlighting their importance in shaping effective experimental results.
Adjusting Annealing Temperature
The annealing temperature holds critical sway over the specificity and efficiency of primer binding to the DNA template. It is the temperature at which the primers anneal—or attach—to their complementary sequences on the target DNA. A well-optimized annealing temperature is crucial for encouraging the formation of stable DNA duplexes while minimizing the chances of non-specific binding that can lead to unwanted amplification products.
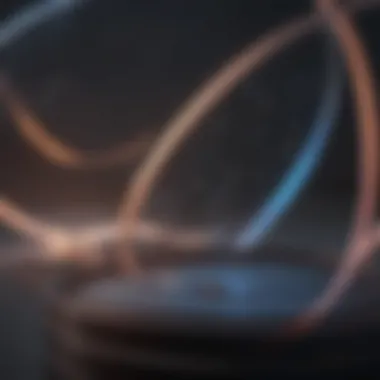
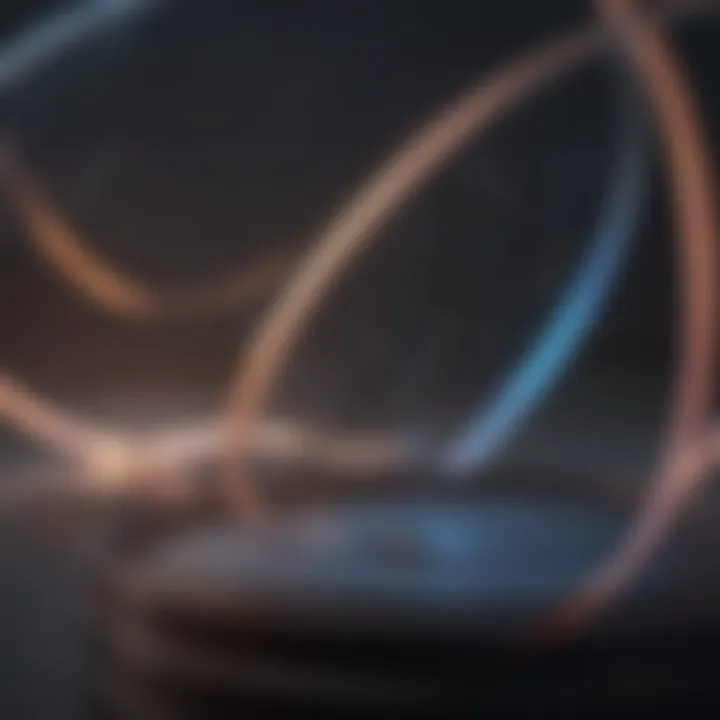
Typically, the optimal annealing temperature tends to be a few degrees lower than the melting temperature (Tm) of the primers used. However, this range can shift based on various factors, such as the primer concentration and the characteristics of the target DNA. As a starting point, many researchers utilize a gradient PCR, which assays a range of annealing temperatures. This approach allows them to pinpoint the most suitable temperature for their specific conditions efficiently.
Having the right annealing temperature prevents issues down the line. A higher temperature might drive away the less stable primer-template hybrids, reducing yield, while a lower temperature could lead to undesired products. It’s all about fine-tuning—the sweet spot between specificity and yield.
Tuning Extension Times
Extension time directly impacts the amount of product synthesized during the PCR process. This phase occurs when the DNA polymerase enzyme synthesizes new DNA strands by adding nucleotides to the primers. The duration of this extension step can vary based on the length of the target DNA and the efficiency of the DNA polymerase in use.
For shorter DNA templates, a quick extension time of around 30 seconds may suffice, while longer sequences might necessitate longer periods, possibly exceeding several minutes. It’s about knowing your template and being aware of the capacity of your enzymes.
For instance, employing high-fidelity DNA polymerases, which often boast superior processivity, allow for shorter extension times without sacrificing product integrity. Adjustments in extension times may be essential if researchers notice low product yields or if they’re after a particularly long amplicon. Experimenting with these variables could save time and resources, enhancing the overall efficiency of the PCR protocol.
Cycling Parameters for Different Applications
Cycling parameters can vary significantly based on the specific needs of the application, whether it be quantification, amplification of particular genes or variations, or detection of pathogens. The general cycling process includes denaturation, annealing, and extension—each step valuable for certain experiments.
In cases of quantitative PCR, or qPCR, it’s essential to adjust these parameters to accommodate real-time data collection. A standard cycle for qPCR may include shorter extension times and multiple cycles, adapting to the quantitative nature of the assay in order to derive numerical insights from the results.
Conversely, for applications such as cloning, it may be necessary to run a larger number of cycles with longer extension times to ensure that sufficient amounts of DNA are produced for manipulation.
Furthermore, incorporating a final extension period can further enhance yield, particularly for longer fragments. Pay attention to over-cycling, though, as it could lead to non-specific products. Understanding the purpose of each PCR experiment steers the decisions made on cycling parameters and leads the way to successful outcomes.
"The art of PCR is as much about the understanding of the variables as it is about the reaction itself; each adjustment leads to a different story in the world of amplification."
"The art of PCR is as much about the understanding of the variables as it is about the reaction itself; each adjustment leads to a different story in the world of amplification."
In summary, optimizing PCR conditions—whether through adjusting annealing temperatures, tuning extension times, or calibrating cycling parameters—significantly enhances the reliability and accuracy of results. A thoughtful approach allows researchers to advance their inquiries and decode the mysteries hidden within the DNA.
Applications of PCR
The versatility of Polymerase Chain Reaction (PCR) is nothing short of remarkable. Its ability to amplify specific DNA sequences has made it a central technique in various fields, bridging the gap between theory and practical application. Understanding these applications is crucial for researchers and professionals, as it demonstrates the breadth of PCR’s impact on science and technology.
Clinical Diagnostics
In the realm of clinical diagnostics, PCR plays a pivotal role. The high sensitivity and specificity of PCR allow for the detection of infectious agents and genetic abnormalities at incredibly low concentrations. For instance, in diagnosing diseases like HIV or tuberculosis, PCR can confirm the presence of pathogens quickly, ensuring timely treatment. This precision minimizes errors that may arise from traditional methods, which might take longer and yield less accurate results.
Furthermore, PCR has significant clinical implications in the field of oncology. It helps in identifying mutations in cancer-related genes, contributing to personalized medicine approaches. In these cases, it’s not just about knowing if a cancer is present; it’s about understanding the specific type of cancer and tailoring treatments accordingly.
"The true strength of PCR in clinical diagnostics lies in its ability to transform the speed and accuracy of disease detection.”
"The true strength of PCR in clinical diagnostics lies in its ability to transform the speed and accuracy of disease detection.”
Research and Development
PCR is also a cornerstone in research and development, across a spectrum of scientific disciplines. It provides researchers with the tools to explore genetic functions, analyze gene expression, and study genetic variations among populations. For example, in molecular biology, scientists utilize PCR to clone DNA fragments, manipulate genes, or create genetically modified organisms.
Many innovative projects in biotechnology, such as the design of new vaccines, heavily rely on PCR techniques. The rapid amplification capabilities of PCR allow researchers to expeditiously generate enough DNA for various experiments, from sequencing to creating recombinant proteins.
Moreover, PCR assists in evolutionary biology, allowing scientists to extract DNA from ancient samples. With techniques like ancient DNA analysis, researchers can explore genetic differences over time and track evolutionary changes that would otherwise remain hidden.
Forensic Science
Forensic science has been revolutionized by PCR technology. In criminal investigations, the ability to analyze minute DNA samples from a crime scene is essential. PCR enables forensic scientists to amplify trace amounts of DNA found on objects of interest, such as clothing or weapons. This amplified DNA can then be used for comparison against databases of known profiles, potentially linking suspects to criminal activities.
However, it is not just about solving crimes; PCR is also used in paternity testing and identifying missing persons. The reliability and accuracy of PCR make it an indispensable tool in the legal system, providing evidence that can stand up in court.
Troubleshooting Common PCR Issues
Troubleshooting PCR is a critical skill for anyone engaged in molecular biology, especially since achieving reliable results is paramount. This section of the article dove into the common challenges that can arise during PCR experiments. Addressing and overcoming these issues not only improves the overall yield and specificity of the PCR products but also saves valuable time and resources.
Understanding how to troubleshoot effectively equips scientists to optimize their experiments and avoid common pitfalls, ensuring that their PCR protocols yield the desired results.
Non-Specific Amplification
Non-specific amplification can be a thorn in the side of many PCR practitioners. It occurs when primers bind to sequences other than the target DNA, leading to the production of undesired products. This can muddy the waters during analysis and interpretation of results.
To address this, consider the following strategies:
- Optimize Annealing Temperature: If the temperature is too low, the primers might bind to non-target sequences. Experiment with shade variation in the annealing temp to find a sweet spot.
- Redesign Primers: If you're facing persistent non-specific binding, it may be time to think about the sequence of the primers. Using software tools to check for potential off-target sites can be beneficial.
- Use Touchdown PCR: This technique begins with a higher annealing temperature that gradually decreases, promoting specificity before allowing more relaxed binding at lower temperatures.
"Identifying and remedying non-specific amplification is akin to clearing the fog from your pathway; it brings clarity to your experimental outcomes."
"Identifying and remedying non-specific amplification is akin to clearing the fog from your pathway; it brings clarity to your experimental outcomes."
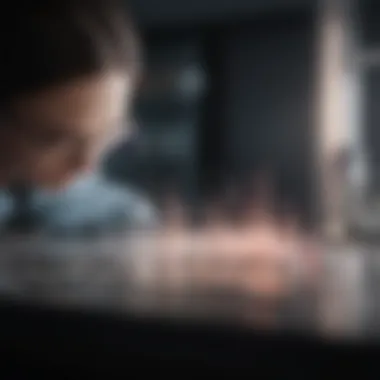
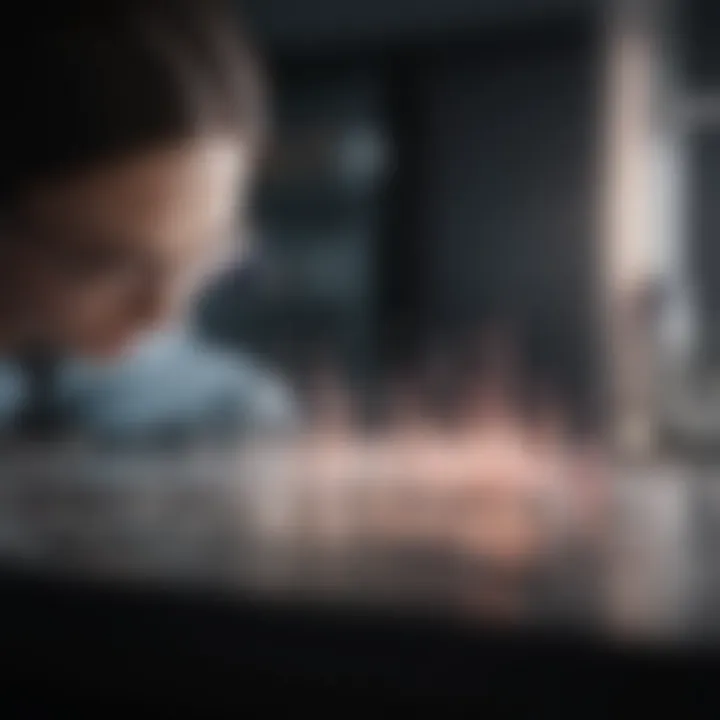
Low Yield of PCR Products
When the PCR reaction fails to produce enough product, it can be incredibly frustrating. Low yields might arise from insufficient template DNA, suboptimal enzyme activity, or issues with primer design. Here are some recommendations to overcome this:
- Increase DNA Template Concentration: It's essential that the amount of template DNA used provides a good starting point. Too little can lead to diminished returns.
- Check the Quality of Reagents: Ensure you're employing high-quality enzymes, buffers, and oligos. Deteriorated reagents can be the cause of poor yield.
- Adjust Extension Times: Sometimes, simply allowing a bit longer for the DNA polymerase to work can yield more product, particularly with long amplicons.
Contamination Problems
Contamination can be the nemesis of PCR, leading to erroneous results and wasted resources. This can come from a variety of sources, such as carryover from previous experiments or contamination from the environment. Here are some useful strategies:
- Strict Sterility Practices: Always employ clean lab techniques. Use dedicated pipette tips, gloves, and work in a clean area.
- Incorporate Negative Controls: This helps in identifying if contamination has occurred. If your PCR yields product in a negative control, something is amiss.
- Utilize Uracil-DNA Glycosylase (UDG): As a powerful tool to prevent carryover contamination, UDG can help degrade any previously amplified products that might be hitching a ride in your samples.
Understanding these troubleshooting techniques can equip researchers with the know-how to swiftly navigate through trial and error in PCR. Ultimately, getting to grips with these challenges can significantly enhance the reproducibility and reliability of your experiments.
Comparative Techniques to PCR
Polymerase Chain Reaction, or PCR, has transformed the landscape of molecular biology, but it isn't the only technique in the toolbox. As scientists drill down into the molecular mechanics of life, they often need a slew of methods to suit specific scenarios. Understanding alternative techniques can not only refine research but also delineate when to use PCR versus other methods. Here, we will explore three pivotal alternatives to PCR: Quantitative PCR, Reverse Transcription PCR, and Digital PCR.
Quantitative PCR (qPCR)
Quantitative PCR, commonly referred to as qPCR, is a twist on the traditional PCR that allows for the quantification of DNA in real-time. This method provides a way to monitor the amplification of DNA during the PCR process, giving a clearer picture of how much of the original DNA template was present.
Benefits of qPCR
- Real-Time Monitoring: Scientists can track the progression of the reaction as it happens, providing immediate feedback on the experiment.
- Quantitative Data: Instead of mere presence or absence, qPCR delivers specific quantifiable data, beneficial for studies in gene expression analysis, viral load measurement, and much more.
- Higher Sensitivity and Specificity: With the ability to detect very low amounts of DNA, qPCR is useful in clinical settings for early diagnosis.
Considerations
- The requirement of specific reagents, like fluorescent dyes, might lead to higher costs.
- Calibration against known standards is necessary for accurate quantification.
Reverse Transcription PCR (RT-PCR)
Reverse Transcription PCR is vital when the target of the amplification is RNA rather than DNA. By converting RNA into complementary DNA (cDNA) using the enzyme reverse transcriptase, researchers set the stage to utilize traditional PCR techniques.
Importance of RT-PCR
- Gene Expression Analysis: This method is widely used to measure mRNA levels—the very basis of gene expression studies.
- Detection of RNA Viruses: RT-PCR is crucial in diagnosing viral infections by detecting the viral RNA, exemplified in the case of SARS-CoV-2.
Key Challenges
- The conversion of RNA to cDNA can be inefficient, affecting the overall yield of the PCR process.
- Selection of high-quality RNA is imperative to avoid issues down the line.
Digital PCR
Digital PCR represents a more recent advancement, carving out a niche by providing absolute quantification of nucleic acids without the need for standard curves. Each molecule of the target DNA is partitioned into a multitude of small reactions, allowing researchers to count the number of positive amplifications directly.
Advantages of Digital PCR
- Precision: Digital PCR can detect rare mutations in a background of wild-type sequences, adding value in fields like oncology, where tumor heterogeneity is a concern.
- No need for standard curves: This feature allows for absolute quantification, making it easier for researchers to report findings reproducibly.
Potential Drawbacks
- The instrumentation required can be expensive and not widely available, limiting its accessibility in certain settings.
- The complexities of designing the experiments can pose a challenge for those new to the technique.
In summary, comparative techniques to PCR like qPCR, RT-PCR, and Digital PCR, provide alternative strategies that can complement traditional PCR methods. Each has its unique strengths and weaknesses, and the choice among them depends on the specific requirements of the experiment at hand. Understanding these alternatives equips researchers with a broader set of tools to tackle molecular biology challenges effectively.
Future Perspectives in PCR Technology
The field of Polymerase Chain Reaction (PCR) is constantly evolving, reflecting the demands and innovations of scientific research. As we delve into the future perspectives in PCR technology, it's essential to recognize several key elements that will undoubtedly shape its landscape. Not only do these advancements offer unique solutions to existing challenges, but they also open new avenues for applications across various fields.
Innovations in PCR Techniques
As technology marches on, PCR isn't left behind. Some of the cutting-edge innovations being explored include:
- Next-Generation PCR (NG-PCR): This method integrates real-time monitoring with next-generation sequencing, allowing for not just the amplification of DNA but also its immediate analysis. The use of fluorescence provides significant benefits in quantifying targets in real-time.
- Microfluidics in PCR: Microfluidic devices have made it possible to conduct PCR in a small, portable format, drastically reducing the amount of reagents needed. This enables more efficient, cost-effective, and scalable solutions, particularly beneficial in resource-limited settings.
- Nanopore PCR: This technique combines PCR with nanopore sequencing, providing rapid results with improved sensitivity and specificity. It's particularly effective in detecting viral and bacterial infections faster than traditional methods.
These innovations support faster diagnostic processes, emphasizing the importance of adaptability in any PCR application. Implementing these technologies could lead to breakthroughs in areas like personalized medicine, where rapid and precise genomic information is crucial.
Applications of Emerging Technologies
The future of PCR will see its methods applied in increasingly inventive ways:
- Point-of-Care Testing: With the rise of portable PCR devices, we can expect more immediate testing environments for infectious diseases. This shift is crucial in public health, especially in remote or underserved regions.
- Environmental Monitoring: PCR technologies can also be applied to assess microbial populations in environmental samples, aiding in pollution detection and biodiversity studies.
- Integrative Genomics: The use of PCR in conjunction with CRISPR technologies can lead to enhanced genome editing capabilities. As the intersections between various gene editing applications and PCR grow stronger, researchers can envision more precise manipulations.
"The advances in PCR techniques are not just about speed; they are about unlocking the potential to analyze our world at an unprecedented scale and depth."
"The advances in PCR techniques are not just about speed; they are about unlocking the potential to analyze our world at an unprecedented scale and depth."
Thus, as we look forward, the continued refinement and innovation in PCR methodologies will play a pivotal role in research development and real-world applications. Scientists and professionals must stay abreast of these changes, integrating novel technologies into their protocols to harness the full power of PCR as an essential scientific tool.