Understanding Gene Expression: Mechanisms and Implications
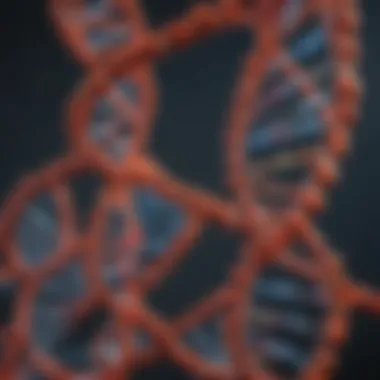

Intro
Gene expression is a fundamental biological process that translates genetic information into functional proteins. Understanding this mechanism is essential for examining how organisms grow, develop, and respond to their environment. The process of gene expression encompasses several stages: transcription, translation, and post-translational modifications. This sequence not only translates DNA into protein but also regulates various physiological functions. In this article, we will explore the intricate mechanisms of gene expression, providing insights into the regulatory factors involved and their implications in health and disease.
Article Overview
Purpose of the Article
The primary objective of this article is to elucidate the intricate workings of gene expression. By analyzing the stages of this process, we aim to shed light on how cells utilize genetic information. Furthermore, it will discuss the regulatory mechanisms that can influence gene expression, affecting everything from cellular functions to entire systems.
Relevance to Multiple Disciplines
Gene expression is not limited to biology alone. Its implications stretch across various fields including medicine, biotechnology, and genetics. For researchers, understanding gene expression helps in identifying potential targets for therapies in genetic disorders. Educators can utilize this information to teach students about the molecular underpinnings of life. As such, this topic holds significance in advancing both research and educational pursuits.
Research Background
Historical Context
The exploration of gene expression has evolved significantly. The foundational work of Gregor Mendel laid the groundwork for understanding heredity. Fast forward to the mid-20th century, the discovery of the DNA double helix by Watson and Crick marked a pivotal point. This was the beginning of deciphering how genes operate. Since then, numerous advancements have taken place, resulting in the current understanding of molecular biology and genetics.
Key Concepts and Definitions
To fully grasp gene expression, it is crucial to define essential terms:
- Transcription: The process where a segment of DNA is copied into RNA.
- Translation: The subsequent step where the RNA is used to synthesize proteins.
- Post-translational Modifications: Chemical changes that occur to proteins after synthesis, influencing their function.
In summary, gene expression involves a complex interplay of molecular mechanisms that are foundational to understanding biological systems. The detailed exploration of this process highlights its critical role in health and disease management, as well as in the advancement of biotechnological innovations.
Understanding Gene Expression
Understanding gene expression is crucial to grasp the fundamental principles of molecular biology. It offers insight into how genetic information is translated into functional products, such as proteins, which perform various roles within cells. This topic touches upon various aspects of biology and medicine, hence its relevance in academic circles.
Gene expression involves multiple stages, including transcription and translation, each defined by intricate mechanisms. By examining these processes, one can appreciate how genetic variability translates to phenotypic diversity. Furthermore, gene expression is pivotal in developmental biology, as it dictates cell differentiation and organismal development.
The practical implications of gene expression extend into fields like genetics and biotechnology. Advances in gene editing and gene therapy hinge on the modulation of gene expression, allowing for targeted treatments in genetic disorders and cancers.
Definition of Gene Expression
Gene expression refers to the process by which information encoded in a gene is used to synthesize a functional gene product, typically a protein. This process starts with transcription, where DNA is transcribed into messenger RNA (mRNA), followed by translation, where the mRNA is decoded to produce a specific protein. The regulation of gene expression is complex and allows cells to respond to internal and external signals by adjusting the amount and timing of protein production.
Historical Context
The understanding of gene expression has evolved significantly since the discovery of DNA's structure by James Watson and Francis Crick in 1953. Initial studies focused on the relationship between DNA and protein, which laid the foundation for the central dogma of molecular biology.
The developments in molecular biology during the latter half of the 20th century unveiled intricate mechanisms of gene control. Notable contributions include the identification of operons in prokaryotes by Francois Jacob and Jacques Monod in the 1960s, illustrating how groups of genes are regulated together. Further advancements in the 1980s, such as the discovery of transcription factors and enhancers, enriched our knowledge about eukaryotic gene expression.
As molecular techniques advanced, the manipulation and understanding of gene expression transformed various biological and medical fields. The synthesis of recombinant proteins and the advent of CRISPR technology represent just a few milestones that have stemmed from our understanding of gene expression.
The Central Dogma of Molecular Biology
The Central Dogma of Molecular Biology serves as a fundamental framework for understanding the processes by which genetic information is transferred within a biological system. This concept highlights the flow of genetic information, primarily from DNA to RNA and finally to proteins. This pathway is central to all life forms, as proteins perform a multitude of functions that are critical to maintaining life.
Each step in the Central Dogma is essential. The first step is transcription, where the genetic code in the DNA is copied to produce messenger RNA (mRNA). This step is crucial because it prepares the genetic code for translation. Without a reliable transcription process, the integrity of the information would be jeopardized.
Next is translation, where the mRNA is decoded to form a specific polypeptide chain, ultimately folding into a functional protein. Proteins are not just structural components; they act as enzymes, hormones, and signal molecules. Their diverse functionalities underscore the significance of the translation phase.
The Central Dogma is not merely a linear pathway. It encompasses various regulatory mechanisms that fine-tune gene expression. Understanding this interplay is crucial in biology, especially in areas such as developmental biology, genetics, and biotechnology.
"The various stages of the Central Dogma illustrate a carefully regulated process that ensures accurate expression of genes, significantly influencing cellular function and ultimately phenotypic attributes."
"The various stages of the Central Dogma illustrate a carefully regulated process that ensures accurate expression of genes, significantly influencing cellular function and ultimately phenotypic attributes."
Also, the handling of ribonucleic acid (RNA), such as different RNA types and their roles, represents an ongoing area of research. Understanding these processes allows for insights into disorders caused by gene expression malfunctions, guiding therapeutic interventions for diseases.
In summary, the Central Dogma is not only a conceptual model but a practical guide. It lays the groundwork for deeper investigations into molecular mechanisms and their implications in broader biological contexts.
From DNA to RNA
The transition from DNA to RNA is a critical initial phase in gene expression. This process, called transcription, involves several key players, including RNA polymerase, which synthesizes RNA strands using the DNA template. The result is an mRNA molecule that mirrors the coding sequence of the gene being expressed.
During transcription, several factors influence how effectively it occurs:
- Promoter Regions: These are specific sequences in DNA where RNA polymerase binds to initiate transcription. Their characteristics dictate how often and efficiently a gene is expressed.
- Transcription Factors: These proteins bind to DNA sequences, enhancing or inhibiting the transcription of specific genes. Their activity is crucial for cellular response to stimuli or developmental cues.
This step ensures the accurate transfer of genetic information, which is vital for the synthesis of proteins that will function as enzymes, structural components, and more in the cell.
From RNA to Protein
Once mRNA is synthesized, it undergoes a process known as translation. This step is where the genetic code is translated into a sequence of amino acids, forming proteins. Translation occurs in the ribosome, a complex machinery that reads the sequence of mRNA.
The process consists of key elements:
- Ribosomes: These are the cellular structures that facilitate the assembly of amino acids into proteins. Ribosomal RNA combines with proteins to form ribosomes, which play a crucial role in the translation.
- tRNA Function: Transfer RNA (tRNA) is responsible for bringing the correct amino acids to the ribosome. Each tRNA molecule has an anticodon that pairs with the correspondingly coded triplet on the mRNA, ensuring the right amino acid is included in the growing protein chain.
- Polypeptide Formation: As tRNA molecules add amino acids one-by-one to the chain, a polypeptide forms. Once completed, this chain undergoes folding and modifications to become a functional protein.
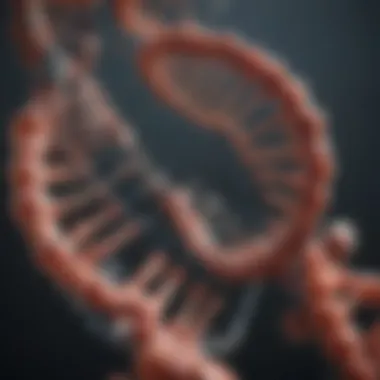
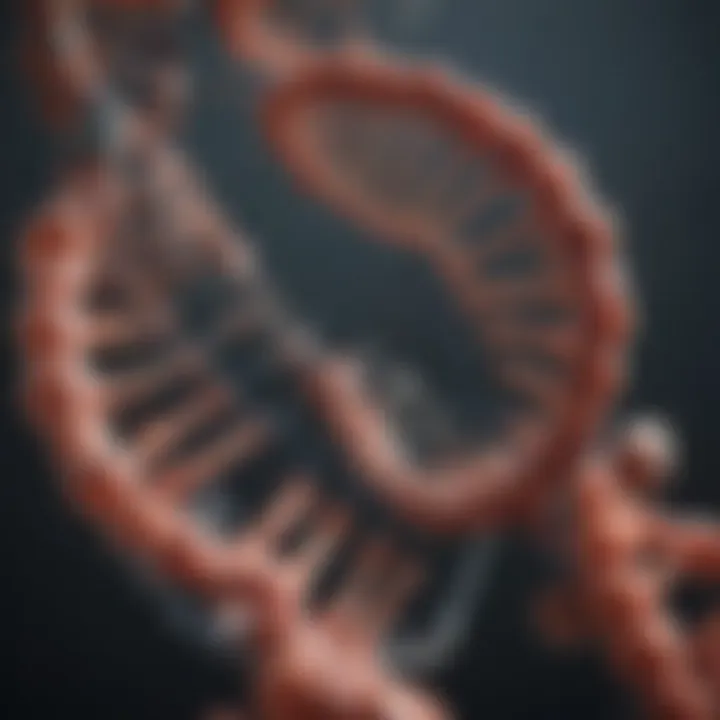
Translation is critical for gene expression, and any errors can lead to nonfunctional proteins, impacting cellular functions and overall organismal health. Understanding this transition not only highlights the nuances of molecular biology but also provides insight into potential therapeutic targets for various diseases linked to protein synthesis abnormalities.
Stages of Gene Expression
Gene expression represents the process through which genetic information in DNA is translated into functional proteins. This process consists of multiple stages, each vital for ensuring that genes are expressed accurately and efficiently. Understanding the stages of gene expression provides insights into how cells operate and respond to their environment. Each stage—transcription, RNA processing, translation, and post-translational modifications—plays a crucial role in determining the final product and its function.
Transcription
Transcription marks the initial step in gene expression, where a specific segment of DNA is transcribed to messenger RNA (mRNA). This process relies heavily on the action of RNA polymerase, which catalyzes the synthesis of RNA from the DNA template. It gathers the necessary nucleotides and assists in forming RNA strands, which serve as templates for further protein synthesis.
Role of RNA Polymerase
The role of RNA polymerase is foundational in facilitating gene expression. It reads the DNA template and synthesizes the complementary RNA strand. A key characteristic of RNA polymerase is its ability to initiate transcription without a primer. This makes it a popular choice for discussions in gene expression.
A unique feature of RNA polymerase is also its various forms in different organisms; for instance, RNA polymerase II is specifically responsible for transcribing mRNA in eukaryotes. Its advantages lie in its versatility and ability to adapt to various gene regulatory mechanisms. However, RNA polymerase may also face challenges, such as issues with transcription fidelity and speed, affecting overall gene expression.
Promoter Regions
Promoter regions are crucial for initiating transcription. These are specific sequences of DNA located upstream of a gene, providing the necessary signals for RNA polymerase to bind and begin the transcription process. One of the key characteristics is that they contain specific motifs recognized by transcription factors, allowing precise control over gene expression. Including promoter regions is a beneficial choice for this article as they illustrate how transcription can be regulated.
A unique feature of promoter regions is their variability in strength and function, which can influence the amount of mRNA produced. Their main disadvantage can be seen in the potential for mutations that might disrupt transcription initiation, leading to reduced gene expression.
Transcription Factors
Transcription factors play a decisive role in the transcription process. They are proteins that bind to promoter regions and other regulatory sequences, facilitating or inhibiting the recruitment of RNA polymerase. The key characteristic of transcription factors is their specificity; each factor often targets certain genes based on cell type and environmental conditions. Discussing transcription factors emphasizes their importance in gene regulation.
A unique feature of these proteins is their complex interactions with one another, forming networks that determine gene expression outcomes. While they offer significant advantages in fine-tuning gene activity, excessive or mistimed transcription factor activity can lead to imbalanced gene expression, which might cause cellular dysfunction.
RNA Processing
After transcription, the precursor mRNA undergoes a series of modifications known collectively as RNA processing. This includes capping, polyadenylation, and splicing. These steps are critical for ensuring that mRNA is mature, stable, and ready for translation.
Capping
Capping refers to the addition of a modified guanine nucleotide to the 5' end of the mRNA strand. This modification protects the mRNA from degradation and facilitates its recognition by ribosomes during translation. Its key characteristic lies in this protective role, and thus it is a beneficial aspect to explore within the article.
Capping also aids in the transport of mRNA from the nucleus to the cytoplasm. However, defects in capping might hinder mRNA stability, affecting overall gene expression outcomes.
Polyadenylation
Polyadenylation involves adding a tail of adenine nucleotides to the 3' end of the mRNA molecule. This process enhances mRNA stability and promotes its export from the nucleus. A notable characteristic of polyadenylation is its influence on mRNA longevity in the cytoplasm, making it relevant for this discussion.
The unique feature of polyadenylation is its role in determining how long mRNA remains intact, ultimately affecting protein synthesis. One disadvantage can be the variability in polyadenylation sites, potentially leading to different protein isoforms.
Splicing
Splicing is the process of removing introns from the precursor mRNA and joining exons together to form a continuous coding sequence. Splicing is significant because it allows for the production of multiple protein variants from a single gene. Its key characteristic is the precision with which it occurs, ensuring that only the relevant portions of mRNA are retained.
Highlighting splicing is advantageous in discussing gene expression, as it underscores the complexity and precision of post-transcriptional modifications. However, errors in splicing can lead to functional deficits or disease, illustrating its importance in maintaining normal cellular functions.
Translation
Translation is the process whereby mRNA is decoded by ribosomes to synthesize proteins. It is a critical link between gene expression and the functional outcomes in the cell.
Ribosomes
Ribosomes are the cellular machinery responsible for protein synthesis, translating mRNA into polypeptide chains. The key characteristic of ribosomes is their dual structure; composed of ribosomal RNA and proteins, they provide the platform for translation. Ribosomes are essential for understanding gene expression, as they directly mediate the assembly of amino acids into proteins.
A unique feature of ribosomes is their capability to function in both prokaryotic and eukaryotic cells but may differ in structure and function. While they are effective for translation, they can encounter issues with translating particularly complex or long mRNA sequences, which might slow the process.
tRNA Function
tRNA (transfer RNA) serves as the adapter molecule during translation. It transports specific amino acids to the ribosome based on the codon sequence in the mRNA. A key attribute of tRNA is its ability to ensure accuracy in matching the correct amino acids to the corresponding codons. This is crucial for the fidelity of protein synthesis, making it an important topic in the article.
The unique feature of tRNA is its structure, which allows it to carry both an anticodon and an amino acid. However, inaccuracies can occur during translation if mischarged tRNA affects the resulting protein, leading to functional consequences.
Polypeptide Formation
Polypeptide formation is the culmination of the translation process, where strings of amino acids are linked together to form proteins. A main characteristic of this stage is the precise sequence of amino acids, as even a single change can significantly impact protein structure and function. Polypeptide formation is a crucial point of focus as it represents the actual synthesis of the product of gene expression.
A unique aspect of polypeptide formation is the potential for post-translational modifications to occur, influencing protein functionality. The challenge can arise from the need for particular conditions for proper folding and assembly, which must be monitored for effective protein function.
Post-Translational Modifications
Post-translational modifications involve further refining and regulating proteins after their synthesis. This stage is essential for ensuring that proteins acquire functional groups necessary for their activity.
Phosphorylation
Phosphorylation is the addition of phosphate groups to proteins, impacting their activity and function. Its key characteristic lies in the rapidity with which it can activate or deactivate protein functions, making it a significant post-translational modification to understand.
Phosphorylation impacts signaling pathways, influencing various cellular responses. However, improper phosphorylation can lead to diseases, underscoring its critical balance in cellular functions.
Glycosylation
Glycosylation is the addition of carbohydrate chains to proteins, which can affect their stability, localization, and interaction with other molecules. A central characteristic of glycosylation is its substantial role in protein function because it can alter a protein’s properties and its recognition by other biomolecules. This provides insight into the diversity of protein functions derived from gene expression.
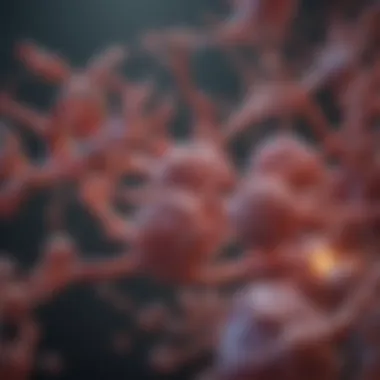
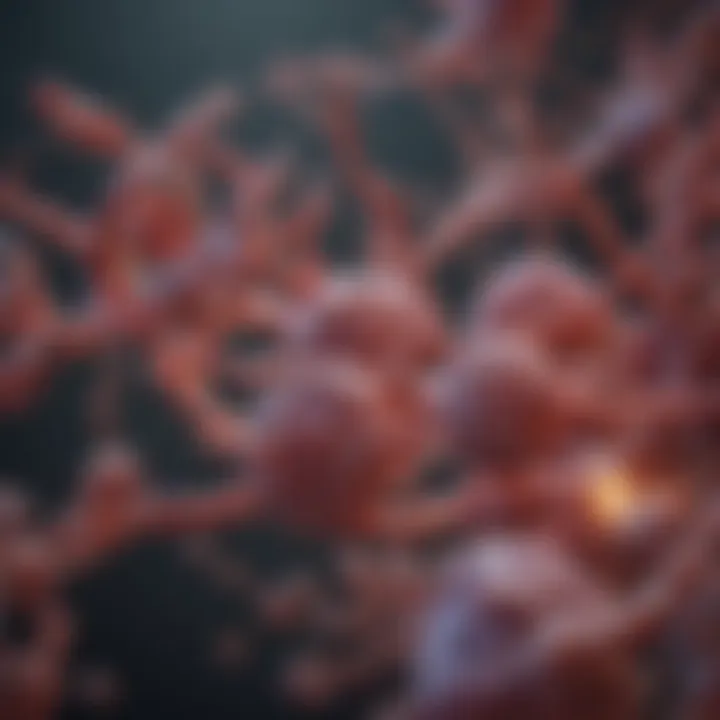
The unique aspect of glycosylation hones in on cellular signaling and immune responses. One downside is the complexity and variability of glycosylation patterns that can complicate the understanding of protein behavior in different contexts.
Proteolytic Cleavage
Proteolytic cleavage involves the enzymatic breaking down of proteins into smaller peptides or amino acids. This process is crucial for activating certain proteins and regulating their degradation. A key characteristic is its capacity to fine-tune protein activity, adding another layer of regulation to gene expression.
Discussing proteolytic cleavage reinforces the idea that the final protein product can be highly regulated. However, uncontrolled cleavage might contribute to pathological conditions, emphasizing the importance of balanced post-translational activities.
Understanding the stages of gene expression provides crucial knowledge about how genetic information is translated into functional outcomes, reflecting the complexity and regulation within biological systems. By exploring each aspect systematically, we can appreciate the seamless integration of these processes and their overarching significance in cellular function.
Regulation of Gene Expression
The regulation of gene expression is a fundamental aspect that determines how genes are turned on or off in different contexts within an organism. This regulation is crucial, as it allows cells to respond appropriately to internal and external stimuli, thus playing a significant role in development, maintenance, and functionality of biological systems. Understanding the mechanisms that govern gene expression regulation provides insights into the complexities of life, including how various factors can influence disease states and therapeutic options.
Transcriptional Regulation
Enhancers
Enhancers are critical regulatory elements in the transcription process. They function by facilitating the binding of transcription factors, which then promote the transcription of nearby genes. One key characteristic of enhancers is their ability to act at a distance, meaning they can regulate genes that are located far away on the same chromosome. This feature makes enhancers popular topics of study as they play a protective role in ensuring that necessary genes are expressed when required. However, enhancers can also be involved in gene misregulation, leading to pathological conditions.
Silencers
Silencers serve to downregulate gene expression by blocking the recruitment of transcription machinery. Similar to enhancers, silencers have a strategic impact by acting at a distance. A significant aspect of silencers is that they provide a mechanism for fine-tuning gene expression. This feature is crucial because it allows cells to balance the need for specific proteins without overexpression, which can lead to adverse effects. However, the complexity of silencer mechanisms can also complicate our understanding and therapeutic strategies in gene regulation.
Transcription Factors
Transcription factors are proteins that help regulate the transcription of specific genes by binding to nearby DNA. They are vital for both activating and silencing changes in expression. A primary benefit of transcription factors is their versatility; they can interact with both enhancers and silencers. This adaptability allows for complex regulatory networks, making the synchrony essential for healthy cellular functions. However, abnormal functioning or mutations of transcription factors can contribute to various diseases, such as cancers.
Epigenetic Modifications
DNA Methylation
DNA methylation is a chemical modification of the DNA molecule, which can impact gene expression without altering the sequence itself. This process usually involves the addition of a methyl group to the cytosine base in the DNA. A key characteristic of DNA methylation is its role in gene silencing; by methylating specific genes, cells can effectively turn them off. This feature is popular in epigenetic studies because it offers a reversible mechanism that can turn genes off in response to environmental changes. However, aberrant DNA methylation patterns are often linked to various diseases, including cancers.
Histone Modification
Histone modification refers to the addition or removal of chemical groups on histone proteins around which DNA is wrapped. These modifications can alter the accessibility of DNA to the transcription machinery. The key characteristic of histone modification is the intricate balance it maintains in gene expression regulation. By modifying histones, cells can either promote or inhibit access to specific genes, thus influencing transcription. While advantageous for cell regulation, improper histone modifications can lead to diseases by contributing to the misexpression of genes.
Non-coding RNAs
Role of miRNA
MicroRNAs (miRNAs) are small non-coding RNA molecules that play significant roles in gene regulation. They function primarily by binding to target messenger RNA molecules, leading to their degradation or blocking their translation. The key characteristic of miRNAs is their ability to fine-tune gene expression levels. This feature is beneficial as it allows for subtle responses to cellular needs and environmental changes. Nevertheless, the dysregulation of miRNAs has been documented in various diseases, highlighting their importance in maintaining healthy cellular functions.
Role of siRNA
Small interfering RNAs (siRNAs) are another class of non-coding RNAs involved in the post-transcriptional regulation of genes. They also operate by binding to complementary RNA sequences and causing their degradation. A notable aspect of siRNAs is their specific targeting capability, which has made them important tools in research and therapeutic applications. They provide a method of silencing genes of interest, particularly in gene therapy contexts. However, the potential off-target effects remain a challenge that requires careful consideration in therapeutic development.
Impact of Gene Expression on Phenotype
The study of how gene expression affects phenotype is essential in understanding biological systems. Phenotype is the observable traits of an organism, which include physical characteristics, behaviors, and physiological properties. Gene expression is crucial because it is the fundamental process by which the information encoded in genes is translated into these traits. Different genes might be expressed in various cell types, developmental stages, or environmental conditions, resulting in phenotypic diversity.
Expression During Development
During development, gene expression orchestrates the formation of tissues and organs from a single cell. Specific genes are activated or silenced at precise times, ensuring proper development paths. This regulation is vital in embryogenesis, where patterns of gene expression guide cells toward differentiation into specific cell types. For instance, genes controlling limb formation are switched on while others are repressed, leading to the emergence of distinct body structures.
Moreover, disruptions in normal gene expression can lead to developmental anomalies. Understanding this process can aid in identifying genetic causes of congenital disorders. By studying model organisms, researchers gain insights into which genes are critical for specific developmental processes, providing a better understanding of human development and associated conditions.
Cell Differentiation
Cell differentiation is closely tied to gene expression. Stem cells have the potential to become any cell type, and this potential is realized through specific patterns of gene activation. Differentiation occurs as genes necessary for a specialized function are expressed while others are downregulated. This selective expression leads cells to develop distinct characteristics and functions.
A classic example of this is the differentiation of stem cells into neurons or muscle cells. Each cell type has a unique set of genes that must be expressed, ensuring they fulfill their roles in the organism's physiology. Moreover, research in this area is allowing scientists to develop regenerative therapies that can reprogram adult cells to become pluripotent, potentially repairing or replacing damaged tissues.
Responses to Environmental Cues
Gene expression is also responsive to environmental signals. Organisms can adapt to changes in their environment through the activation of specific genes. For example, in response to temperature changes, some plants express heat shock proteins to help cope with stress. Likewise, in humans, exposure to UV light prompts the expression of genes involved in melanin production, leading to tanning of the skin as a protective response.
These adaptive mechanisms highlight the flexibility of gene expression. They reveal how phenotypes can be adjusted based on external conditions, ensuring the survival and reproduction of the organism. The ability to respond to environmental cues is crucial in evolutionary terms, allowing species to thrive under varying conditions.
Understanding gene expression offers profound insights into the adaptability of organisms and their development.
Understanding gene expression offers profound insights into the adaptability of organisms and their development.
Thus, the impact of gene expression on phenotype is multifaceted. It encompasses developmental processes, cell specialization, and environmental interactions. By delving deeper into these areas, researchers continue to uncover the genetic basis of phenotypic variation, paving the way for advances in medicine and biotechnology.
Gene Expression in Disease
Gene expression plays a crucial role in the development of various diseases, influencing both the onset and progression of these conditions. Understanding how gene expression is altered in disease contexts provides insight into the underlying mechanisms that contribute to health and illness. This section highlights how aberrant gene expression can lead to significant health issues, exploring specific diseases where gene expression plays a critical role.
Cancer and Aberrant Gene Expression
Cancer is one of the most studied areas in the context of gene expression. At its core, cancer can be viewed as a consequence of deregulated gene expression leading to uncontrolled cell proliferation. Specific genes, known as oncogenes, can drive the transformation of normal cells into cancerous ones when expressed at high levels or activated inappropriately. Conversely, tumor suppressor genes, which normally function to restrain cell growth, may become inactivated, further promoting cancer development.
Key gene alterations include mutations in genes such as TP53, which is pivotal in regulating the cell cycle and apoptosis. When TP53 is mutated, cells can evade programmed cell death, allowing them to survive longer and proliferate without constraints.
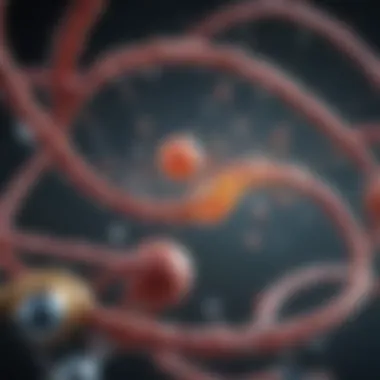
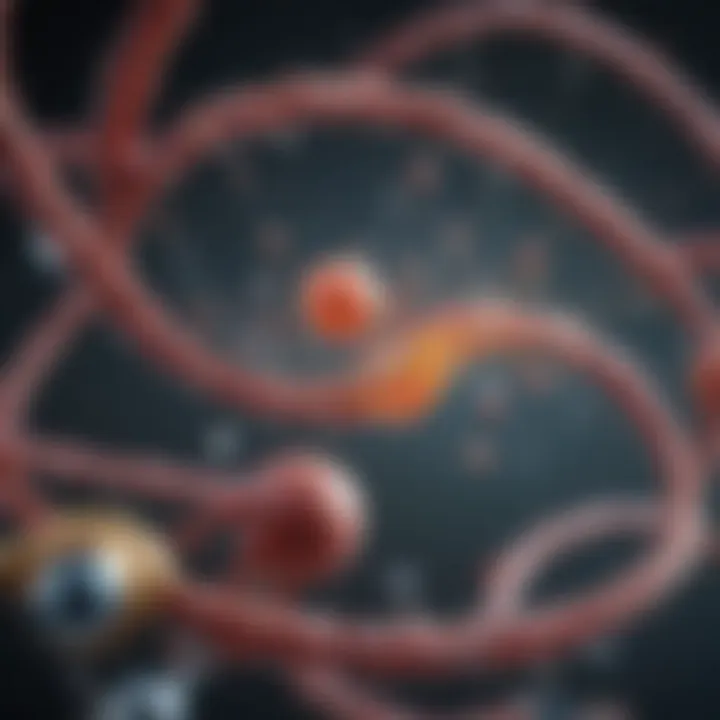
"Understanding gene expression in cancer allows for targeted therapies, which can significantly improve patient outcomes."
"Understanding gene expression in cancer allows for targeted therapies, which can significantly improve patient outcomes."
Moreover, epigenetic changes such as DNA methylation can silence important tumor suppressor genes, contributing to malignant behavior. The dynamic nature of gene expression in cancer highlights the complexity of treating such diseases, as therapies must account for the variability in gene expression across different patients.
Genetic Disorders
Genetic disorders arise from defects in genes, which may be due to mutations affecting the normal expression levels of these genes. Conditions such as cystic fibrosis, sickle cell anemia, and Huntington's disease illustrate this. In cystic fibrosis, for example, the CFTR gene is altered, leading to defective chloride channels. This defect results in the production of thick mucus, causing various physiological complications.
Often, these genetic disorders illustrate the direct consequence of gene expression changes. Studies show that understanding the abnormalities in gene expression related to these conditions is vital for developing effective treatment strategies and genetic counseling.
Furthermore, advancements in gene therapy, targeting specific genetic mutations, are now being explored to correct these expression issues at their source, providing hope for patients affected by these disorders.
Infectious Diseases
Infectious diseases also reflect the significance of gene expression in health. Pathogens can manipulate host gene expression to create an environment favorable for their survival. Viruses are particularly adept at this; they inject their genetic material into host cells, hijacking cellular machinery to express viral proteins essential for their replication. This often disrupts normal cellular processes, leading to disease.
For instance, the interaction of the HIV virus with host T cells leads to significant changes in gene expression, weakening the immune response and paving the way for opportunistic infections. Understanding these gene expression dynamics enables researchers to develop antiviral therapies that can effectively combat these infections.
Biotechnological Applications
Biotechnological applications of gene expression are transforming multiple fields, particularly medicine, agriculture, and environmental science. These advancements hinge on our understanding of how genes are expressed and regulated. The relevance of this topic in the article is manifold, providing insights into novel therapeutic methods and advancements in genetic engineering.
The integration of biotechnology into healthcare has been profound, especially with gene therapy approaches. These techniques have made it possible to treat genetic disorders by delivering healthy copies of genes to replace mutated ones. Notably, specific methods like viral vectors are designed to carry the therapeutic genes into the target cells, leading to significant improvements in a patient’s health. However, challenges such as effective delivery and potential immune responses must be addressed to optimize these treatments.
The potential applications of biotechnology extend to CRISPR and genome editing. This groundbreaking tool has revolutionized the precision with which genetic material can be edited. Unlike previous methods, CRISPR allows targeting specific DNA sequences, making it possible to modify genes with unmatched accuracy. This can lead to correcting genetic mutations responsible for diseases, enhancing crop resilience, and facilitating innovative research. Ethical considerations surrounding CRISPR use, particularly in humans, have sparked important debates, necessitating careful oversight.
In addition, advancements in synthetic biology have opened new frontiers in biotechnology. Synthetic biology focuses on designing and constructing new biological parts, devices, and systems, or redesigning existing biological systems for useful purposes. Applications range from biofuel production to developing organisms capable of synthesizing pharmaceuticals. This field combines engineering principles with biological knowledge to create organisms with tailored functionalities. It also raises biosafety concerns, as engineered organisms must be carefully assessed for their impact on ecosystems and human health.
In summary, the role of biotechnological applications in gene expression is critical. From novel gene therapies to innovative editing techniques and the burgeoning field of synthetic biology, these applications hold the potential to reshape numerous aspects of our lives. The harnessing of gene expression mechanisms paves the way for breakthroughs that may one day alleviate human suffering and enhance our understanding of biological processes.
"The future of biotechnology is shaped by our growing understanding of gene expression and its regulation."
"The future of biotechnology is shaped by our growing understanding of gene expression and its regulation."
Each of the highlighted biotechnological applications is crucial in addressing some of the most pressing challenges facing society today. As research progresses, continuing to explore these frontiers is essential for unlocking the potential of genetic understanding.
Research Frontiers in Gene Expression
The field of gene expression research is continuously evolving, revealing insights that are crucial to understanding biology at a cellular and molecular level. By identifying the mechanisms involved, researchers can uncover the nuances of gene regulation and the roles genes play in health and disease. This section discusses recent advances that propel our comprehension forward, including single-cell profiling, long non-coding RNAs, and the microbiome's involvement.
Single-Cell Gene Expression Profiling
Single-cell gene expression profiling is a revolutionary technique that allows scientists to analyze the gene expression of individual cells. This method differs from traditional bulk analysis, which averages signals from many cells, often obscuring important cell-to-cell variations. By using next-generation sequencing technologies like RNA sequencing, researchers can capture the unique expression profiles of thousands of single cells.
The importance of this technique lies in its potential to identify rare cell types and subpopulations that play significant roles in biological processes and diseases. For instance, in cancer research, understanding the heterogeneity within tumors can inform treatment strategies tailored to distinct cell populations. Moreover, single-cell profiling provides insights into developmental biology by tracking differentiation processes in real-time.
Some recent advancements in this area include improved methods for cell isolation and computational tools that can analyze the resulting data efficiently. This continues to be a vibrant area of research, yielding valuable discoveries about cellular function, interactions, and the overall dynamics of gene expression.
Long Non-coding RNAs
Long non-coding RNAs (lncRNAs) are emerging as crucial regulators of gene expression, challenging the traditional view that only coding genes are significant in cellular functions. Unlike messenger RNAs, which translate into proteins, lncRNAs can regulate gene expression at various levels, including transcription, RNA stability, and translation.
Research has shown that lncRNAs can function as molecular scaffolds, bringing together proteins and other molecules to regulate target genes. For example, the lncRNA XIST plays a vital role in X-chromosome inactivation in females, demonstrating how these molecules can influence developmental processes.
The implications of understanding lncRNAs are substantial. They may serve as potential biomarkers for diseases, including cancer, where their dysregulation is frequently observed. By targeting lncRNA pathways, novel therapeutic strategies can be developed. Therefore, continued exploration of lncRNAs presents a compelling research frontier that may unlock new aspects of gene regulation.
Role of the Microbiome
The microbiome, the diverse community of microorganisms inhabiting various environments within our body, substantially influences gene expression. These microorganisms interact with host physiology and can impact health by modulating immune responses, metabolism, and even behavior.
Recent studies provide evidence that microbial communities can regulate host gene expression and affect pathways associated with diseases such as obesity, diabetes, and autoimmune disorders. The composition of the microbiome can lead to changes in the host’s gene expression profiles, which may enhance or inhibit certain metabolic functions.
Understanding the intricate relationship between the microbiome and gene expression opens the door to novel therapeutic strategies, including probiotics and dietary interventions. It suggests a level of complexity in health that requires further exploration, combining microbiome research with traditional gene expression studies. This interdisciplinary approach has the potential to drive significant advancements in personalized medicine and treatment strategies.
Closure
Understanding gene expression is crucial to comprehending the fundamental processes of biology. The mechanisms through which gene expression occurs not only shape organismal development but also influence the intricate dynamics of health and disease. By elaborating on the stages of gene expression, such as transcription, RNA processing, and translation, this article paints a detailed picture of how genetic information is utilized within cells.
The importance of gene expression can be viewed from several perspectives. Firstly, it underpins many biological processes, including cell differentiation and response to environmental stimuli. The ability to regulate gene expression allows organisms to adapt to changing conditions, ensuring survival and functionality within diverse environments. Additionally, the link between aberrant gene expression and various diseases highlights the significance of accurate regulation in maintaining physiological balance. Examples like cancer and genetic disorders exemplify how misregulation can lead to dire health consequences.
In terms of biotechnology, the exploration of gene expression opens new avenues for gene therapy, CRISPR technology, and synthetic biology. These advancements not only hold promise for treating diseases but also for enhancing agricultural practices and biomanufacturing processes. The knowledge garnered from studying gene expression mechanisms serves as a foundation for innovative applications aiming to improve the quality of life.
In summary, grasping the complexities of gene expression equips researchers, students, and professionals with significant insights that echo throughout modern biology, medicine, and biotechnology.
"Gene expression is the bridge connecting the genetic code to the functional entities influencing life's myriad forms."
"Gene expression is the bridge connecting the genetic code to the functional entities influencing life's myriad forms."
Summary of Key Points
- Gene expression is the process where information from a gene is used to synthesize functional gene products, typically proteins.
- Central processes include transcription, RNA processing, translation, and post-translational modifications.
- Regulation of gene expression can occur through several mechanisms, including transcription factors and epigenetic modifications.
- Aberrations in gene expression are implicated in numerous diseases, notably cancer and genetic disorders.
- Innovations in biotechnology leverage understanding of gene expression for therapeutic and agricultural advancements.
Future Directions
The exploration of gene expression is far from complete, with multiple avenues ripe for research.
- Single-cell sequencing will facilitate the understanding of gene expression at the individual cell level. This could further elucidate heterogeneity within tissues and among cell populations.
- The investigation of long non-coding RNAs could provide new insights into regulatory networks influencing gene expression.
- Understanding the microbiome's role in gene expression may reveal how gut flora impacts host health and disease susceptibility.
As we delve deeper into the complexities surrounding gene expression, it remains imperative to consider ethical implications and ensure responsible advancement in biotechnological applications. Emerging findings offer vast potential, calling for interdisciplinary collaboration to maximize their impact.