Delta G Primer: Understanding Gibbs Free Energy
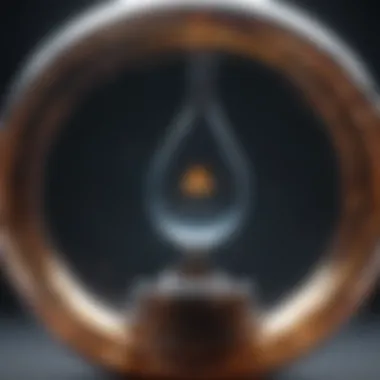
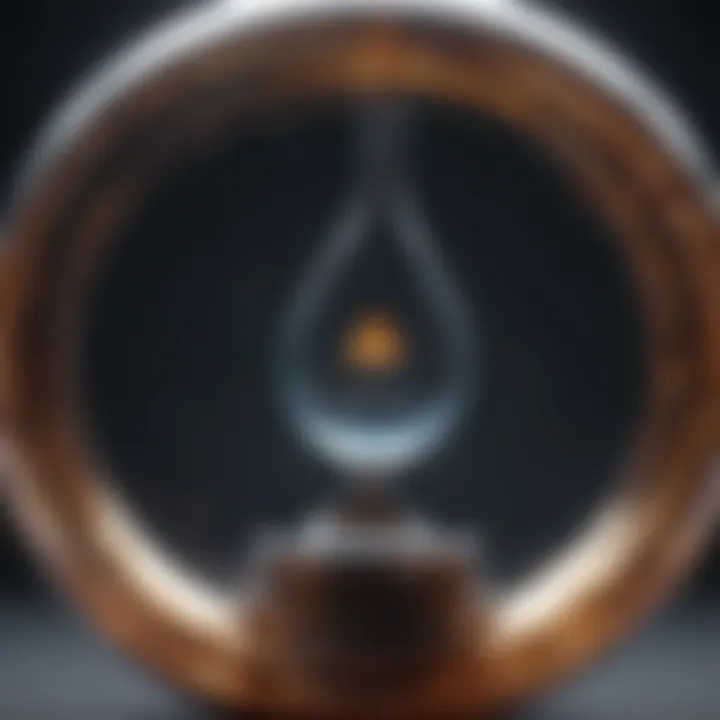
Article Overview
Understanding Gibbs Free Energy, commonly referred to as Delta G, is essential for anyone engaged in scientific research or education. This article aims to provide a detailed exploration of Delta G, the mathematical principles that govern it, and its applications across various disciplines, including chemistry and biology.
Purpose of the Article
The primary purpose of this article is to clarify what Gibbs Free Energy is, its significance in predicting the spontaneity of chemical reactions, and how it plays a crucial role in thermodynamic processes. By delving into its implications, the article strives to enhance the reader's understanding of these complex topics. This guide is structured to construct a solid foundation for students, researchers, and professionals who wish to grasp the importance of Gibbs Free Energy in their work and studies.
Relevance to Multiple Disciplines
Gibbs Free Energy finds relevance in multiple scientific disciplines. In chemistry, it helps predict reaction spontaneity, guiding chemists in developing new materials and understanding natural processes. In biology, it underpins metabolic pathways, explaining how organisms convert energy. Its applications extend to environmental science, engineering, and even economics, where energy considerations play a pivotal role in decision-making.
Research Background
A solid grasp of the historical context and key concepts surrounding Delta G is crucial for understanding its significance today.
Historical Context
The concept of Gibbs Free Energy emerged in the late 19th century, credited primarily to the physicist Josiah Willard Gibbs. This framework evolved as part of the broader development of thermodynamics, which traditionally dealt with heat, work, and energy transformations. Gibbs' contribution was instrumental in bridging the gaps between macroscopic phenomena and atomic or molecular interactions, enabling a more profound insight into chemical and physical processes.
Key Concepts and Definitions
Gibbs Free Energy (G): It is defined as the energy associated with a chemical reaction that can be used to do work at constant temperature and pressure. The equation for calculating Gibbs Free Energy is:
[ G = H - TS ]
Where:
- G is Gibbs Free Energy,
- H is enthalpy,
- T is temperature in Kelvin,
- S is entropy.
A reaction is spontaneous if the change in Gibbs Free Energy (ΔG) is negative. Conversely, a positive ΔG indicates that the reaction requires energy input to proceed.
"A deeper understanding of Gibbs Free Energy provides essential insights into both chemical spontaneity and the nature of reversible reactions."
"A deeper understanding of Gibbs Free Energy provides essential insights into both chemical spontaneity and the nature of reversible reactions."
Prologue to Delta G
The exploration of Delta G, or Gibbs Free Energy, offers profound insights into thermodynamics. It serves as a cornerstone for understanding energy changes within chemical processes. The significance of Delta G extends beyond mere calculations, as it masks a wealth of information about reaction spontaneity and equilibrium conditions. Understanding this concept is vital for students, researchers, and professionals engaged in chemistry and related fields.
Defining Gibbs Free Energy
Gibbs Free Energy is a thermodynamic quantity that represents the maximum reversible work obtainable from a thermodynamic system at constant temperature and pressure. It is denoted as ( G ), where the change in Gibbs Free Energy is represented as ( \Delta G = G_final - G_initial ). A negative value of ( \Delta G ) indicates that a process can occur spontaneously, whereas a positive value implies non-spontaneity under the given conditions. This relationship between ( \Delta G ), enthalpy, and entropy is crucial for predicting the feasibility of reactions. The underlying theory combines principles from chemistry and physics, illustrating how energy is conserved and transformed.
Historical Context
The term "Gibbs Free Energy" is named after the American scientist Josiah Willard Gibbs, who introduced this concept in the 19th century. Gibbs's insights arose during the development of thermodynamics as a scientific discipline. His work laid the foundation for modern chemistry and physics, bridging gaps between theoretical and practical applications of energy. Before Gibbs's contributions, various energy concepts existed, but they were often disconnected and lacked a unified framework. Today, the work of Gibbs is seen as a turning point that helps explain both natural and industrial processes.
Understanding historical context enhances one’s appreciation for how thermodynamic principles have evolved. It highlights the collaborative nature of scientific progress, where ideas build on one another, leading to modern applications in various fields such as chemistry, biology, and engineering.
Theoretical Foundation of Delta G
The theoretical foundation of Delta G is crucial in understanding the role of Gibbs Free Energy in thermodynamics. This foundation provides the necessary principles and mathematical tools to assess the energy changes during chemical reactions. By exploring these elements, one gains insights into the spontaneity of reactions, equilibriums, and various practical applications in different scientific domains.
Thermodynamic Principles
Thermodynamic principles serve as the backbone of the Gibbs Free Energy concept. These principles are derived from the laws of thermodynamics, which outline how energy is transferred in systems. At the core, the First Law asserts that energy cannot be created or destroyed, only transformed. This lays the ground for evaluating how energy is stored as Gibbs Free Energy.
The Second Law introduces the concept of entropy, a measure of disorder in a system, and highlights that natural processes tend to move towards a state of increased disorder. This law directly ties to Gibbs Free Energy since the change in Gibbs Free Energy ( ( \Delta G )) during a reaction relates to changes in enthalpy and entropy. Understanding these principles allows one to predict whether a reaction is spontaneous or non-spontaneous under specified conditions, setting the stage for deeper analysis.
Mathematical Representation
Mathematics is the language through which theoretical concepts are expressed quantitatively. In the context of Delta G, the mathematical representation is essential for calculating Gibbs Free Energy and its implications in chemical reactions.
Basic Equation of Gibbs Free Energy
The basic equation of Gibbs Free Energy is given as:
[ \Delta G = \Delta H - T \Delta S ]
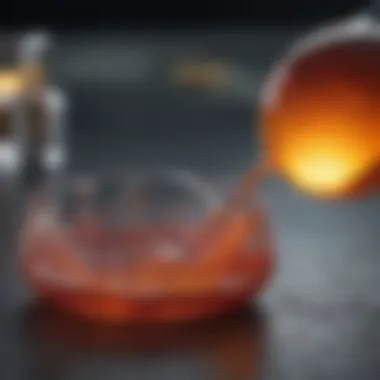
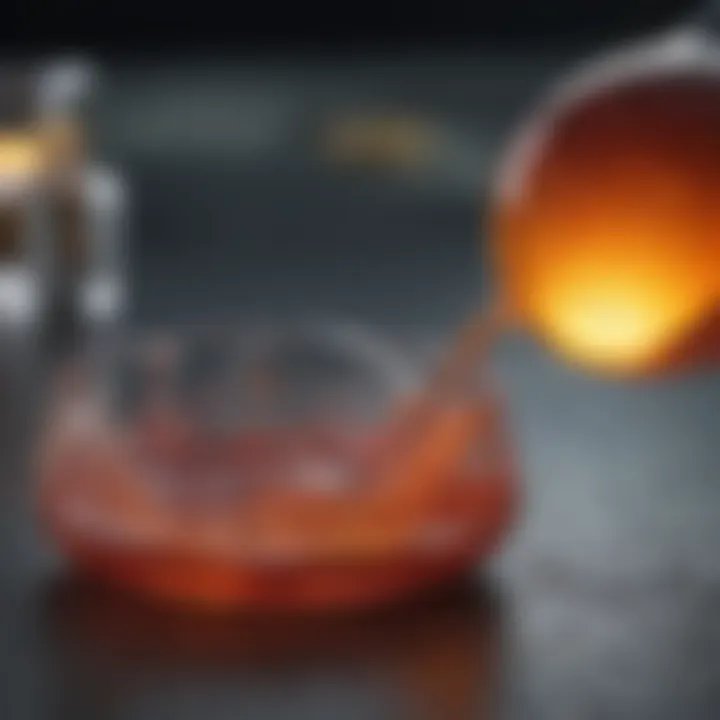
In this equation, ( \Delta H ) represents the change in enthalpy, ( T ) is the absolute temperature in Kelvin, and ( \Delta S ) denotes the change in entropy. This equation is valuable for predicting the spontaneity of reactions. For instance, if ( \Delta G ) is negative, the reaction can occur spontaneously. The standout feature of this equation is its clarity; it succinctly combines energy changes with system temperature and disorder. This compact form is particularly advantageous for both introductory studies and advanced research at various levels of chemistry.
However, the equation does have limitations. It assumes constant pressure and temperature, which may not hold in all practical scenarios. Thus, while the equation provides foundational insights, one must be cautious when applying it to complex systems.
Relation to Enthalpy and Entropy
The relation of Gibbs Free Energy to enthalpy and entropy is fundamental in understanding thermodynamic processes. Enthalpy reflects the heat content in a reaction, while entropy measures disorder. The interplay between these two states gives rise to the total Gibbs Free Energy of a system.
This relationship is crucial because as systems change, reactions can become favorable or unfavorable. For instance, in exothermic reactions, a negative ( \Delta H ) can drive a reaction towards spontaneity even when entropy does not significantly change. Conversely, in endothermic processes where ( \Delta S ) is positive, the reaction may still proceed if the temperature is sufficiently high to make the ( T \Delta S ) term outweigh ( \Delta H ).
The unique feature of this relationship is that it offers predictive power; knowing the signs of ( \Delta H ) and ( \Delta S ) gives a clear understanding of the behavior of reactions. Nevertheless, there are disadvantages, particularly in the interpretation of reactions under non-standard conditions.
Understanding the theoretical foundation of Delta G is vital for analyzing chemical processes and predicting outcomes in complex systems.
Understanding the theoretical foundation of Delta G is vital for analyzing chemical processes and predicting outcomes in complex systems.
Interpreting Delta G Values
Understanding Delta G values is crucial for analyzing chemical reactions and thermodynamic processes. Delta G, or Gibbs Free Energy, directly relates to the spontaneity and favorability of a reaction. By interpreting these values, researchers can predict whether a reaction will occur spontaneously under specified conditions. This insight not only aids in thermodynamic studies but also enhances practical application in various fields including chemistry, biology, and material science. A comprehensive interpretation lays the groundwork for effective experimentation and innovation.
Spontaneity of Reactions
Negative Delta G
A negative Delta G value indicates that a reaction is spontaneous under the given conditions. This characteristic is essential in thermodynamics because it indicates that the system, when it transitions from reactants to products, releases energy. One key feature of negative Delta G is its ability to drive reactions forward without the need for external energy input. This benefit is fundamental for many biological processes, such as cellular respiration.
The downside, however, lies in the fact that not all reactions with a negative Delta G are quick to occur. The activation energy may still be high, leading to slower reaction rates despite the favorable energy state. Thus, while negative Delta G values suggest spontaneity, it does not necessarily imply that the reaction will proceed rapidly.
Positive Delta G
Conversely, a positive Delta G value reflects a non-spontaneous reaction under normal conditions. In this case, energy must be supplied to convert reactants into products. This value is relevant in chemical equilibria, where the system tends to shift to minimize energy input,
Positive Delta G reactions are crucial in various biochemical processes like photosynthesis, where energy from sunlight is absorbed. The unique feature of positive Delta G reactions is that they indicate the need for external energy sources, which can be vital for certain industrial applications or synthetic processes. A major disadvantage of relying on positive Delta G is that it increases complexity in managing energy inputs, often requiring careful planning in practical applications.
Zero Delta G
A zero Delta G signifies that a reaction is at equilibrium. In this state, the rate of the forward reaction equals the rate of the reverse reaction, meaning there is no net change in products or reactants. This characteristic is particularly useful for understanding chemical equilibria and optimizing reaction conditions effectively.
The unique feature of a zero Delta G condition arises from the balance it provides. It offers insights into how changes in concentration, temperature, or pressure might shift the equilibrium point. However, this condition also reflects a lack of driving force, as no energy is available for further reaction progression. Hence, while an equilibrium state provides stability, it often requires external intervention to initiate any desired change in the system.
Standard Conditions and Their Importance
In thermodynamics, standard conditions refer to a set of specified parameters under which thermodynamic measurements are taken. These typically include standard pressure (1 atm) and standard temperature (25°C). The significance of these conditions lies in their ability to provide a consistent framework for analyzing Gibbs Free Energy and other thermodynamic properties.
When discussing Delta G, using standard conditions allows scientists to compare results across different experiments and systems reliably. Calculating Delta G values under standard conditions serves as a reference point, enabling researchers to predict how systems will respond to changes. This is crucial in both laboratory settings and industrial applications, where understanding reaction feasibility is paramount. By establishing a common ground, standard conditions enhance the accuracy and applicability of thermodynamic principles across various fields.
Factors Affecting Delta G
Understanding the factors that influence Gibbs Free Energy, or Delta G, is essential for grasping the nuances of thermodynamic processes. This knowledge not only aids in predicting the behavior of chemical reactions but also deepens insights into various scientific realms, including chemistry, biology, and material science. The significance of Delta G stems from its role in determining the spontaneity of a reaction, as well as its dependence on external conditions. Several key factors that affect Delta G include temperature, concentration, and pressure. Each of these factors can alter the Gibbs Free Energy, thereby impacting reaction predictions and outcomes.
Temperature Effects
Temperature plays a crucial role in influencing Gibbs Free Energy. In many situations, raising or lowering the temperature can shift the Gibbs Free Energy of a reaction. The relationship between Delta G and temperature is primarily governed by the equation:
[ \Delta G = \Delta H - T \Delta S ]\
In this equation, (\Delta H) represents the change in enthalpy, (T) is the absolute temperature in Kelvin, and (\Delta S) denotes the change in entropy. A higher temperature can increase the value of (-T \Delta S), potentially turning a positive (\Delta G) into a negative one, which signifies a spontaneous reaction. Conversely, at lower temperatures, the enthalpy change may dominate, affecting the spontaneity.
Concentration Effects
Concentration of reactants and products is another significant factor in determining Delta G. The change in Gibbs Free Energy is dependent on the concentrations of reactants and products in equilibrium. According to the reaction quotient, the relationship can be expressed as:
[ \Delta G = \Delta G^\circ + RT \ln Q ]\
Here, (Q) represents the reaction quotient, while (R) is the universal gas constant. As the concentration of the reactants increases or decreases, the value of (Q) shifts, consequently impacting the Gibbs Free Energy. An increase in reactant concentration often results in lowering (\Delta G), making the reaction more spontaneous. Conversely, high concentrations of products can raise the Gibbs Free Energy, hindering spontaneity.
Pressure Contributions
Pressure is particularly significant for reactions involving gases. Changes in pressure can influence the Gibbs Free Energy by shifting the equilibrium state of a reaction. For gaseous systems, the effect of pressure is governed by the Ideal Gas Law and can affect the concentration of gaseous reactants and products. An increase in pressure can favor the direction of a reaction that produces fewer gas molecules, thus altering the Delta G.
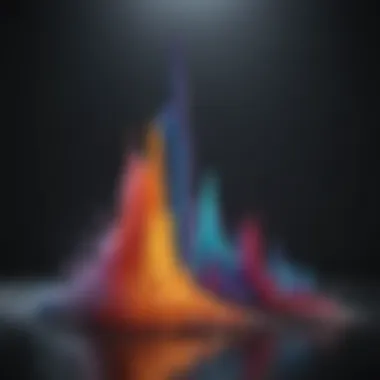
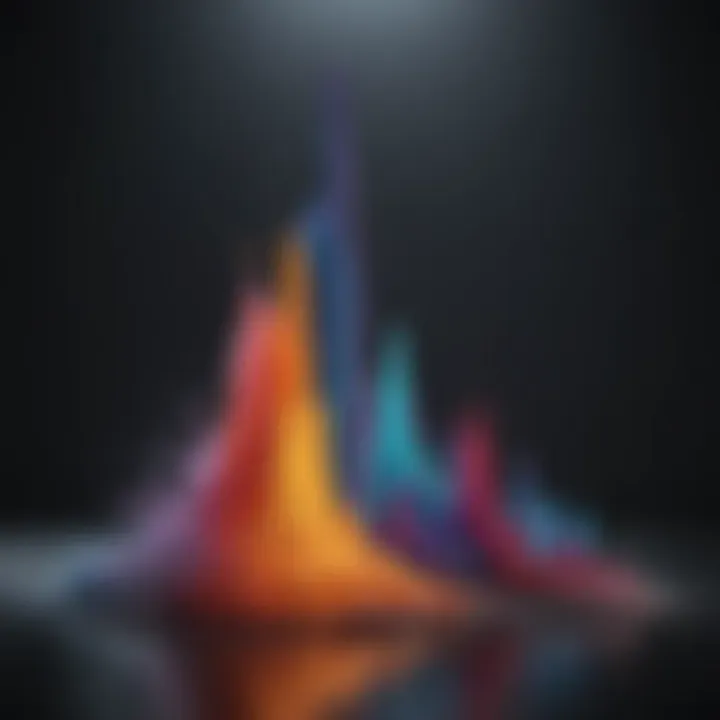
In summary, temperature, concentration, and pressure are interrelated aspects that significantly affect the Gibbs Free Energy of a system. A comprehensive understanding of these factors is not just academically enriching; it is also fundamentally practical for applications in chemical and biological sciences.
It is essential to remember that the interplay between these factors can determine the feasibility and direction of chemical reactions. Understanding these relationships enhances our capability to manipulate conditions for desired outcomes in various scientific fields.
It is essential to remember that the interplay between these factors can determine the feasibility and direction of chemical reactions. Understanding these relationships enhances our capability to manipulate conditions for desired outcomes in various scientific fields.
Applications of Delta G in Chemistry
Gibbs Free Energy, denoted as Delta G, plays an essential role in chemistry. It provides a framework to understand how and why reactions occur. The significance of Delta G is multifaceted, influencing a diverse range of applications from reaction dynamics to equilibrium considerations. By evaluating Gibbs Free Energy, chemists can determine whether a reaction will proceed spontaneously under given conditions. This ability is crucial for designing experiments and optimizing processes in various fields including biochemistry, material science, and chemical engineering.
Understanding Delta G helps in predicting the efficiency of chemical reactions. In practical terms, it allows scientists to select suitable reactants or alter conditions to favor the formation of desired products. Through this understanding, researchers can enhance yield, minimize waste, and streamline reactions.
Chemical Reaction Dynamics
Chemical reaction dynamics refers to how reactions occur and the rates at which they proceed. Delta G aids in dissecting these dynamics by allowing chemists to analyze the thermodynamic feasibility of reactions. The concept of activation energy also ties into this framework. While a reaction may have a negative Delta G, indicating spontaneity, the actual transformation may be slow. This is where kinetics enters the picture.
For instance, the hydrolysis of ATP, which is a negative Delta G process, is vital for cellular energy transfer. Despite its energetic favorability, the reaction rate is modulated by enzymes. In studying reactions, both Delta G and reaction kinetics must be integrated to give a full picture of the dynamics involved, as they impact how quickly products can form.
Equilibrium vs. Kinetics
The relationship between equilibrium and kinetics is fundamental to understanding many chemical processes. Delta G plays a critical role in this discussion. At equilibrium, the Gibbs Free Energy of the system is minimized. In this state, the rates of the forward and reverse reactions are equal, leading to a stable concentration of reactants and products.
However, kinetics focuses on the rate at which these equilibrium states are reached. This distinction is essential. A reaction might have a favorable Delta G, yet if the kinetics are unfavorable, it may take a long time to reach equilibrium. This aspect is crucial for many chemical applications.
To succinctly summarize:
- Delta G determines if a reaction can occur spontaneously.
- Kinetics refers to the speed of reactions.
- Both parameters are needed to fully understand chemical processes.
"Gibbs Free Energy provides a lens through which the energetic favorability and the kinetic accessibility of reactions can be assessed, essential for both theoretical explorations and practical applications in chemistry."
"Gibbs Free Energy provides a lens through which the energetic favorability and the kinetic accessibility of reactions can be assessed, essential for both theoretical explorations and practical applications in chemistry."
Biological Significance of Delta G
Understanding the biological significance of Delta G, or Gibbs Free Energy, is vital for grasping how energy transformations affect living systems. At the core of cellular processes, Delta G plays a crucial role in determining whether biochemical reactions will proceed spontaneously. This section delves into the fundamental aspects of metabolic reactions and how they relate to cellular energetics.
Metabolic Reactions
Metabolic reactions involve intricate networks of enzyme-catalyzed processes that govern the conversion of nutrients into energy. These reactions are essential for maintaining homeostasis in various organisms. The Gibbs Free Energy change ( ( \Delta G )) for metabolic reactions indicates whether a reaction will occur energetically favorably. Generally, metabolic reactions can be classified as exergonic or endergonic.
- Exergonic Reactions: These reactions result in a negative Delta G, which signifies a release of energy. Respiration, the process cells use to extract energy from glucose, is a prime example. In cellular respiration, glucose is oxidized, leading to energy production necessary for ATP synthesis.
- Endergonic Reactions: In contrast, reactions that yield a positive Delta G require energy input to proceed. An example of this is the synthesis of glucose during photosynthesis, where plants absorb sunlight to convert carbon dioxide and water into glucose.
Understanding these transformations helps clarify how organisms utilize and manage energy to facilitate life processes.
Energetics of Cellular Processes
The energetics of cellular processes is tightly linked to the concept of free energy. Cellular processes, such as cellular respiration and biosynthesis, often require precise energy management. Delta G informs cells on how to allocate and utilize available energy effectively.
For instance, during ATP hydrolysis, the energy released drives various endergonic reactions within the cell. This coupling of reactions is crucial for metabolic pathways, ensuring that energy produced in exergonic reactions is harnessed for necessary cellular work. Additionally, the regulation of Delta G in biological systems highlights the importance of enzyme activity and metabolic regulation in maintaining a balance between energy supply and demand.
"Biological systems rely on the principles of Gibbs Free Energy to create order from chaotic environments while supporting life at molecular levels."
"Biological systems rely on the principles of Gibbs Free Energy to create order from chaotic environments while supporting life at molecular levels."
In summary, the biological significance of Delta G extends beyond mere thermodynamic principles. It provides a framework for understanding the energy dynamics that sustain life. Whether in metabolic reactions or the energetics of various cellular processes, Delta G proves to be an indispensable concept in biochemistry and molecular biology.
Real-World Examples of Delta G
Understanding real-world applications of Delta G offers insights into how this concept functions beyond theoretical frameworks. It highlights the relevance and practicality of Gibbs Free Energy in various contexts, enriching our appreciation of its implications in both chemistry and biology. By examining specific examples, we can delineate how Delta G informs our comprehension of systems at equilibrium as well as those driven toward spontaneity under certain conditions.
Case Study: ATP Hydrolysis
A prominent case study of Delta G in action is the hydrolysis of adenosine triphosphate (ATP). ATP is often known as the energy currency of the cell. Its hydrolysis—a breakdown reaction where ATP is converted to adenosine diphosphate (ADP) and an inorganic phosphate (Pi)—is a key process in cellular metabolism. The standard free energy change (ΔG°) for this reaction is approximately -30.5 kJ/mol. The negative value signifies that the reaction is spontaneous under standard conditions.
The energy released during ATP hydrolysis is harnessed by the cell to facilitate various biological functions. These include muscle contraction, nerve impulse propagation, and the synthesis of macromolecules. In this context, understanding Delta G not only illustrates the energetics of cellular processes but also sheds light on energy transfer mechanisms within biological systems. The principle of energetics can be observed here: ATP hydrolysis releases sufficient energy to drive endergonic reactions, highlighting the utility of Delta G in maintaining cellular functions.
Example: Combustion Reactions
Combustion reactions also serve as significant examples of Delta G’s role in real-world scenarios. When we consider the combustion of hydrocarbons, such as methane (CH₄), we see a clear application of Gibbs Free Energy in predicting whether the reaction will occur spontaneously.
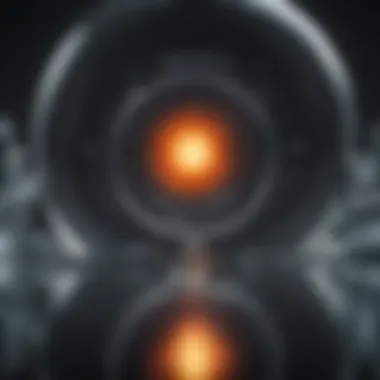
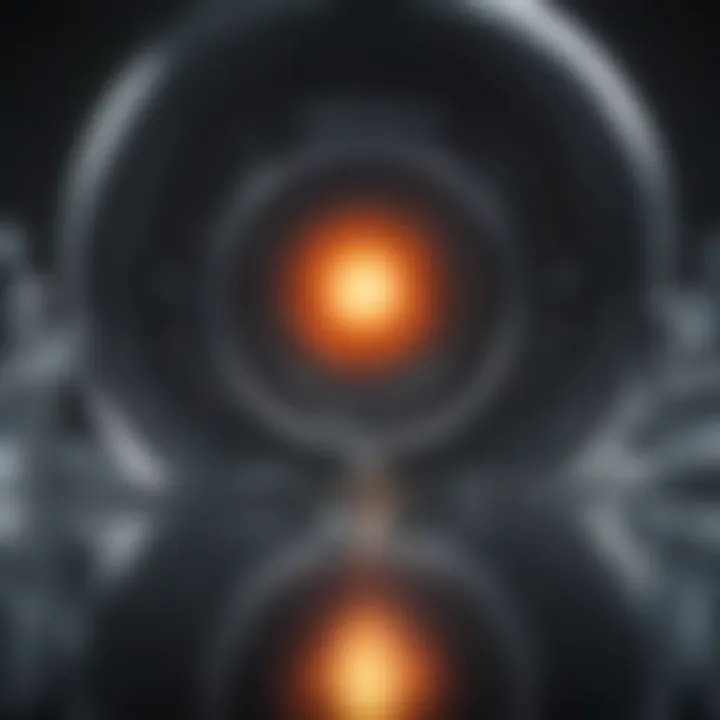
The overall reaction for the combustion of methane can be simplified as follows:
[ CH₄ + 2O₂ → CO₂ + 2H₂O ]
The ΔG for this reaction is negative at standard conditions, indicating that combustion occurs spontaneously and releases energy in the form of heat and light. This generation of energy is what drives many industrial applications, including power generation and internal combustion engines in vehicles. Moreover, understanding the Gibbs Free Energy associated with combustion reactions is essential for optimizing fuel efficiency and reducing emissions—critical aspects of current environmental challenges.
Both examples emphasize how Delta G has practical implications in our daily lives, from how our cells produce energy to how we power our vehicles. They reinforce the importance of this thermodynamic concept in navigating both biological processes and technological advancements.
Current Research Trends Involving Delta G
Understanding the current research trends involving Delta G reveals its significance in various scientific fields. This section delves into two main areas: advancements in computational chemistry and the applicability of Delta G in material science. The exploration of these trends demonstrates how Delta G can influence research projects, providing essential insights into thermodynamic processes.
Advancements in Computational Chemistry
Computational chemistry has made significant strides in the past few years, allowing researchers to simulate and predict the behavior of molecules with remarkable accuracy. Delta G plays a crucial role in these simulations. By calculating Gibbs Free Energy, scientists can determine the thermodynamic feasibility of reactions. This ability allows for optimization of reaction conditions and the design of novel chemical pathways.
Recent advancements include more sophisticated algorithms and increasingly powerful computational resources. These tools enable the calculation of Delta G under various conditions, yielding dynamic insights into chemical reactions. For instance, machine learning models are now being integrated into computational chemistry to enhance the predictive capabilities regarding Gibbs Free Energy. This integration helps in assessing potential reaction mechanisms and their associated energy changes efficiently.
Additionally, big data analytics is being utilized to aggregate findings from numerous studies. Such analytics point to trends in Gibbs Free Energy changes across different reaction types. The combination of these innovations in computational chemistry supports more strategic research approaches, ultimately driving progress in the field.
Delta G in Material Science Applications
In material science, Delta G is increasingly recognized as a pivotal parameter in understanding phase behavior and stability of materials. The ability to manipulate Gibbs Free Energy is essential in developing new materials with desirable properties. As material science seeks to innovate in areas such as nanotechnology and biomaterials, the implications of Delta G grow larger.
Researchers leverage Delta G to tailor material synthesis processes. For example, in the development of catalysts, knowing the Gibbs Free Energy helps in selecting appropriate materials and conditions for optimized performance. Furthermore, misscalculations related to Delta G can lead to failures in material design, making accurate Gibbs Free Energy calculations critical.
Finale
Exploring the current trends related to Delta G demonstrates its indispensable role in advancing knowledge across various fields. Both computational chemistry and material science benefit significantly from the insights that accurate Gibbs Free Energy calculations provide. Focusing on these trends leads to a deeper appreciation of thermodynamics and its applications in modern research.
Challenges and Limitations of Using Delta G
Understanding the challenges and limitations associated with Gibbs Free Energy, or Delta G, is crucial for making informed scientific decisions. While Delta G provides valuable insights into thermodynamic processes, it has inherent constraints that must be recognized. These challenges can influence the interpretation of results and even the applications of Delta G in real-world scenarios. A clear grasp of these limitations arm researchers and students with the necessary context to avoid misinterpretations that may arise from oversimplified conclusions.
Assumptions in Calculations
When calculating Delta G, several assumptions underlie the process, potentially affecting the accuracy of results. Key assumptions often include:
- Ideal Behavior: Many calculations assume ideal conditions, which rarely occur in practical situations. Real substances behave according to specific interactions and forces, which can skew calculated predictions.
- Constant Temperature and Pressure: Delta G calculations typically assume constant temperature and pressure. However, in many reactions, especially biological systems, temperature and pressure fluctuations can profoundly affect outcomes.
- Equilibrium State: It is assumed that systems reach an equilibrium state before measuring Delta G. Misjudgments regarding when equilibrium is achieved can lead to significant errors in the Gibbs Free Energy values.
It is essential to be aware of these assumptions to interpret data accurately, especially in complex chemical reactions.
Complex Systems Analysis
Complex systems in thermodynamics often include various interacting components, such as multiple reactions and phases. Here are several aspects in which Delta G faces challenges:
- Non-Ideality: In real-life systems, interactions between particles (like solvation or partial ionic dissociation) can affect the free energy in non-linear ways. Delta G calculations may oversimplify these complex interactions.
- Multistep Processes: In processes that occur in multiple steps, the cumulative effect on Gibbs Free Energy needs to be considered. Each step may not contribute equally, and ignoring intermediate steps can lead to faulty conclusions.
- Environmental Influences: Factors like pH, ionic strength, and the presence of catalysts (or inhibitors) can influence Delta G but are not always accounted for in basic models. This oversight may lead to misrepresentation of the spontaneity of certain reactions.
"Understanding the limitations of Delta G is as vital as the concept itself. Ignoring these challenges can lead to misapplications of thermodynamic principles."
"Understanding the limitations of Delta G is as vital as the concept itself. Ignoring these challenges can lead to misapplications of thermodynamic principles."
By recognizing these limitations, researchers can approach their analyses of Gibbs Free Energy with a more critical mindset, enhancing the quality of their experimental design and theoretical interpretations.
End and Future Directions
The conclusion will synthesize the key points discussed in the article about Gibbs Free Energy, emphasizing its significance in thermodynamics, and outline future research directions in this field. Understanding Delta G is essential for comprehending reaction spontaneity and the energetics involved in chemical processes. The article aims to clarify complex thermodynamic concepts, offering insights that are valuable for both academic and practical applications.
Recap of Key Concepts
Gibbs Free Energy, or Delta G, is a thermodynamic parameter that determines the favorability of a reaction at constant temperature and pressure. The primary points to recap include:
- Definition and Importance: Delta G quantifies the energy available to do work during a process, allowing predictions about whether a chemical reaction will occur spontaneously.
- Mathematical Foundation: It is defined mathematically as ( Delta G = Delta H - T imes Delta S ), where (Delta H) is enthalpy change, (T) represents temperature in Kelvin, and (Delta S) is entropy change.
- Interpretation of Values: A negative delta G indicates spontaneity, while positive or zero values suggest non-favorability or equilibrium, respectively.
- Factors Influencing Delta G: Changes in temperature, concentration, and pressure can greatly affect the Gibbs Free Energy, impacting both chemical reactions and biological processes.
- Applications Across Disciplines: Beyond chemistry, Delta G principles extend into biology, materials science, and engineering, underscoring its broad relevance in scientific research.
Potential Areas for Further Research
Exploring future directions in the study of Gibbs Free Energy can lead to significant advances in our understanding of thermodynamics. Some promising areas of research include:
- Non-Traditional Thermodynamic Environments: Investigating Gibbs Free Energy in non-standard conditions, such as extreme temperatures or pressure, can provide insights into processes such as supercritical fluid behavior.
- Integration with Quantum Mechanics: The relationship between quantum states and thermodynamic functions may yield deeper insights into energy landscapes and reaction mechanisms.
- Applications in Renewable Energy: Analyzing Gibbs Free Energy could enhance the efficiency of energy conversion systems, like fuel cells and batteries, contributing to sustainable technology.
- Complex Biological Systems: Further research could examine how Delta G influences metabolic pathways, providing valuable information that could advance medical or biotechnological applications.
- Machine Learning in Thermodynamics: Employing machine learning algorithms to predict Delta G under various conditions can result in optimized reaction pathways or novel synthetic routes.
By focusing on these areas, researchers can significantly advance the field, leading to innovative applications that address some of the most pressing challenges in science today.
By focusing on these areas, researchers can significantly advance the field, leading to innovative applications that address some of the most pressing challenges in science today.
In summary, the exploration of Gibbs Free Energy is vital for accelerating advancements across disciplines. Understanding its role will facilitate deeper engagement with both theoretical and practical aspects of thermodynamics.