Transmission Genetics: Principles and Applications
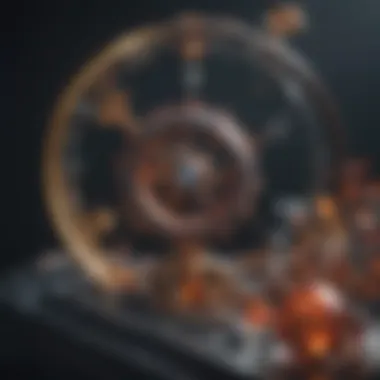
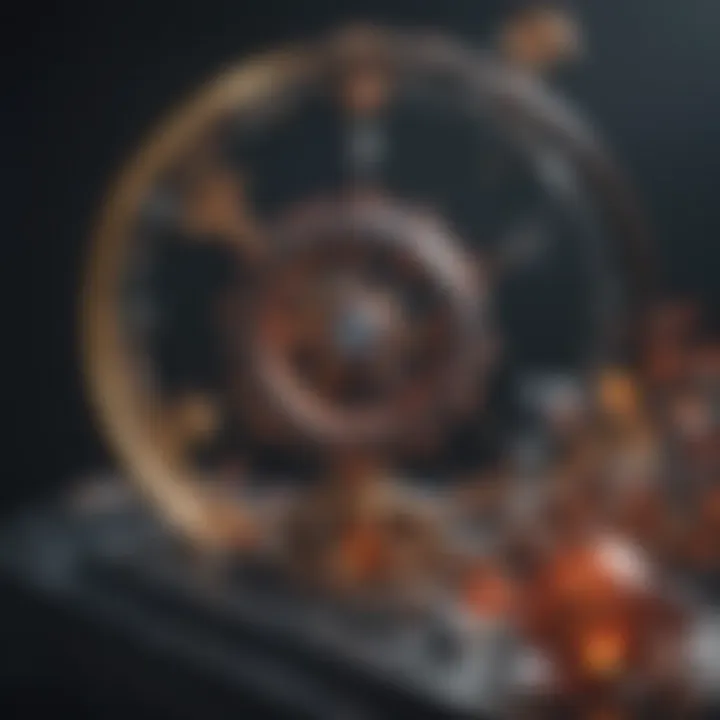
Intro
Transmission genetics is a specialized field that focuses on the ways in which genetic information is passed from one generation to the next. Understanding these principles is crucial for various scientific disciplines including medicine, agriculture, and genetics itself. This article explores both foundational elements and emerging applications of this vital area of study.
The mechanisms of heredity have long fascinated researchers and contribute to critical advancements in technology and biology. From Gregor Mendel's pea plants to recent discoveries in genomic editing, the journey through transmission genetics reveals influential concepts that shape our understanding of heredity and variation.
Article Overview
Purpose of the Article
The goal of this article is to illuminate the principles of transmission genetics and its contemporary relevance. It aims to provide a clear perspective on how genetic traits are inherited and the implications of these traits in real-world applications. By bridging historical insights with current methodologies, the article seeks to enhance the comprehension of this complex yet essential field.
Relevance to Multiple Disciplines
Transmission genetics transcends traditional boundaries, impacting several fields:
- Medicine: Understanding hereditary diseases and potential genetic therapies.
- Agriculture: Enhancing crop yields through selective breeding and genetic modification.
- Evolutionary Biology: Analyzing genetic variation to understand species adaptation and survival.
This broad applicability demonstrates how crucial the study of transmission genetics is across various scientific endeavors.
Research Background
Historical Context
The study of genetics began in the 19th century with Gregor Mendel, who established the basic laws of inheritance. His work laid the foundation for modern genetics, providing a framework that scientists still reference today. Subsequent developments, including the discovery of DNA structure by James Watson and Francis Crick, further propelled genetic research into the modern era.
Key Concepts and Definitions
Key concepts in transmission genetics include:
- Alleles: Different forms of a gene that can lead to variations in traits.
- Genotype: The genetic constitution of an individual, which may influence observable characteristics.
- Phenotype: The physical expression of a genotype, often influenced by both genetic and environmental factors.
Understanding these concepts is essential for exploring more advanced topics, such as gene mapping and genetic engineering. Each concept serves as a building block for the next level of inquiry in the genetics field.
"The inheritance of traits follows patterns that have been documented and refined through years of research, highlighting the complexities of genetic information."
"The inheritance of traits follows patterns that have been documented and refined through years of research, highlighting the complexities of genetic information."
Further exploration of transmission genetics can yield insights into our future and drive innovations across multiple sectors.
Prelude to Transmission Genetics
Transmission genetics is a critical area within genetic research that examines how traits and characteristics are passed from one generation to the next. This fundamental concept is essential for understanding not only basic biological processes but also the implications of genetic inheritance in various sectors, including medicine and agriculture. Grasping the principles of transmission genetics enriches our appreciation of heredity and variation among organisms.
Definition and Scope
Transmission genetics focuses on the mechanisms of genetic inheritance. At its core, it examines the roles played by genes, alleles, and chromosomes in passing on traits. The scope of transmission genetics encompasses several facets:
- Mendelian inheritance: This area outlines the basic laws governing heredity and is primarily grounded in the experimental work of Gregor Mendel, who established foundational principles based on pea plant studies.
- Non-Mendelian inheritance: This examines more complex patterns of inheritance that do not fit Mendel's laws, including incomplete dominance, codominance, and polygenic traits.
- Applications: The implications of transmission genetics extend beyond basic science to practical uses in medicine, such as understanding genetic disorders and in agriculture, for developing improved crop varieties.
This area of study provides frameworks for predicting inheritance patterns and offers insights into genetic variability, essential for breeding programs and genetic counseling.
Historical Background
The roots of transmission genetics can be traced back to the mid-19th century with Gregor Mendel's pioneering work on pea plants. Mendel’s meticulous experiments led to the formulation of his laws of inheritance, which laid the foundation for classical genetics. However, the recognition of Mendel's contributions did not occur until the early 20th century. It was only after the rediscovery of his work that scientists began to appreciate the significance of his findings.
Subsequent advancements in the understanding of chromosomes and genes marked significant milestones. The development of the chromosome theory of inheritance further solidified the link between genetics and heredity. In the decades that followed, researchers such as Thomas Morgan expanded upon Mendelian principles through their work with fruit flies, establishing more complex patterns of inheritance.
Over the years, technological advancements, including the discovery of DNA's structure by Watson and Crick in 1953, revolutionized the field and paved the way for molecular genetics. Today, transmission genetics continues to evolve, integrating insights from biotechnology and genomics, and remains an essential component of various scientific disciplines.
"Understanding transmission genetics is vital for appreciating the complexities of heredity and its impact on health and agriculture."
"Understanding transmission genetics is vital for appreciating the complexities of heredity and its impact on health and agriculture."
Fundamental Principles of Genetics
The fundamental principles of genetics are essential to understanding the mechanisms that govern heredity and variation in all forms of life. In this section, we will delve into the core concepts that provide a framework for transmission genetics. These principles explain how genetic traits are passed on from parents to offspring, illuminating the intricate processes behind biological inheritance. A clear grasp of these principles sets the stage for further exploration of both Mendelian and non-Mendelian inheritance, offering valuable insights into genetic diversity and fitness across populations.
Genes and Alleles
Genes are segments of DNA that serve as the basic units of heredity. Each gene contains the instructions for building proteins, which are crucial for cell function and development. Within a gene, variations exist in the form of alleles. Alleles can be classified as dominant or recessive. A dominant allele will manifest its trait even if only one copy is present. In contrast, a recessive allele only expresses its trait when two copies are present.
The understanding of genes and alleles is pivotal in genetics. It allows researchers and practitioners to predict outcomes of genetic crosses and understand the potential for certain traits to appear in future generations. For example, in pea plants, Mendel discovered that the allele for purple flowers is dominant over the allele for white flowers, leading to predictable ratios in offspring phenotypes. This foundational knowledge is crucial for fields such as agriculture and medicine, where predicting genetic outcomes can lead to better crop yields or the understanding of hereditary diseases.
- Key Points about Genes and Alleles:
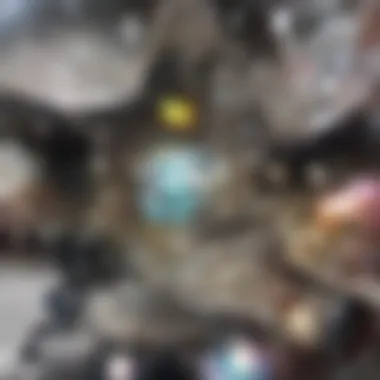
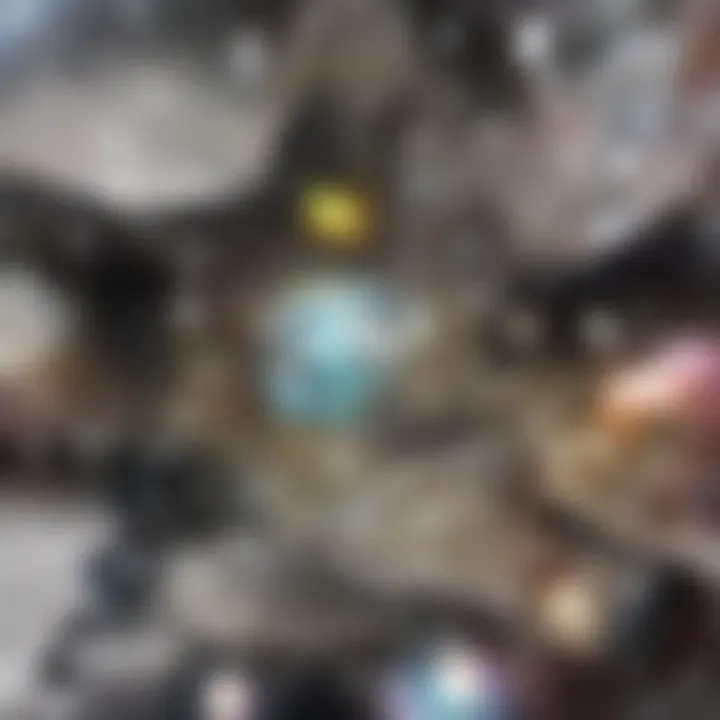
- Genes dictate specific traits and functions in organisms.
- Alleles contribute to genetic variation within a species.
- Dominance relationships determine how traits are expressed in individuals.
Genotype vs Phenotype
In genetics, understanding the difference between genotype and phenotype is vital. The genotype refers to the genetic makeup of an organism, containing all the alleles that the organism possesses. The phenotype, on the other hand, is the observable expression of these genes, influenced not only by genotype but also by environmental factors.
This distinction is important in studies of biology for several reasons. First, it helps clarify how specific genetic combinations lead to observable characteristics in organisms. For example, two organisms may have the same genotype yet display different phenotypes due to variations in environmental conditions, such as nutrition or temperature.
Moreover, genotype and phenotype are critical concepts in research, breeding, and medicine. Understanding these terms allows geneticists to analyze how traits are inherited and how they respond to environmental changes. In plant and animal breeding, knowledge of phenotype helps breeders select organisms that exhibit desirable traits, thereby improving or stabilizing characteristics in future generations.
"The relationship between genotype and phenotype is complex, involving interactions between genes, environment, and chance."
"The relationship between genotype and phenotype is complex, involving interactions between genes, environment, and chance."
In summary, the exploration of fundamental principles of genetics equips us with the necessary tools to navigate the complexities of transmission genetics. Through understanding genes, alleles, genotype, and phenotype, we build a foundation for recognizing the broader implications these concepts have across various fields, including agriculture, medicine, and evolutionary biology.
Mendelian Inheritance
Mendelian inheritance is a cornerstone of transmission genetics. It provides a clear framework for understanding genetic inheritance patterns observed in many organisms. Named after Gregor Mendel, who discovered the fundamental laws of heredity, this area of study delves into how traits are passed down from one generation to the next.
Mendel's work laid the groundwork for modern genetics by identifying how specific traits segregate within offspring. This allows researchers to predict inheritance patterns based on parental genotypes. Understanding Mendelian inheritance is vital not only for genetics but also for various practical applications in areas such as agriculture and medicine.
Mendel's Laws of Inheritance
Mendel proposed three key principles of inheritance: the law of segregation, the law of independent assortment, and the law of dominance. Each principle underpins how genetic traits are transmitted through generations.
- Law of Segregation: This law states that during the formation of gametes, alleles for a trait separate so that each gamete carries only one allele for each gene.
- Law of Independent Assortment: According to this law, alleles of different genes assort independently of one another during gamete formation. Thus, the inheritance of one trait will not affect the inheritance of another.
- Law of Dominance: Mendel found that in cases where two different alleles are present, one allele may mask the expression of another. This means that a dominant allele can overshadow a recessive one in determining phenotype.
"Mendel's laws provide a simplified model that helps in predicting the outcomes of genetic crosses, acting as a foundation for further studies in genetics."
"Mendel's laws provide a simplified model that helps in predicting the outcomes of genetic crosses, acting as a foundation for further studies in genetics."
These laws have substantial implications, informing breeding strategies in agriculture and enhancing our understanding of genetic disorders in healthcare.
Punnett Squares and Predictions
Punnett squares are a powerful tool for visualizing the expected outcomes of genetic crosses based on Mendel's principles. This method helps in calculating the probability of inheriting particular traits, making it extremely useful in both research and practical applications.
To create a Punnett square, one first identifies the genotypes of the parents. Each cell of the square then represents a possible genotype of the offspring.
- Steps to create a Punnett square:
- Determine the alleles of both parents.
- Set up the square by placing one parent's alleles along the top and the other parent's alleles along the side.
- Fill in the squares to show potential offspring genotypes.
Using Punnett squares allows for clear predictions about traits, aiding in breeding programs and education. They simplify complex genetic concepts into a comprehensible visual format, facilitating better understanding and communication in genetics.
Non-Mendelian Inheritance Patterns
Non-Mendelian inheritance patterns provide crucial insights beyond the classical Mendelian framework. While Mendel's laws lay the foundation of genetics, they only account for certain types of traits and their inheritance. Non-Mendelian patterns explore the complexities of genetic inheritance that do not adhere strictly to Mendel's principles. Understanding these patterns is essential in various fields such as medicine and agriculture, where traits are subject to multiple alleles and environmental influences. This section will elaborate on three significant types of non-Mendelian inheritance: incomplete dominance, codominance, and polygenic inheritance.
Incomplete Dominance
Incomplete dominance occurs when the phenotypic expression of a heterozygous genotype is distinct from and often intermediate to those of the homozygous genotypes. This pattern blurs the lines of dominance, leading to a new phenotype that is a blend of the two parental traits. For example, in the case of snapdragon flowers, crossing a red flower (RR) with a white flower (WW) results in pink flowers (RW), showcasing this blending effect. This phenomenon is not just limited to floral color; it applies to various traits in other organisms as well.
Importance
The understanding of incomplete dominance helps in predicting phenotypic ratios that differ from the expected Mendelian ratios. It also provides a framework for research into traits that show similar patterns, thereby enhancing breeding practices. It sheds light on how traits can express themselves in more nuanced ways than traditional genetics might suggest.
Codominance
Codominance is a form of inheritance where both alleles in a heterozygous individual fully express themselves without blending. An exemplary case is observed in blood type inheritance; for instance, in the AB blood type, both A and B alleles are expressed equally, resulting in a phenotype that shows characteristics of both alleles. This is in stark contrast to incomplete dominance, where traits blend into a new form
Benefits
Understanding codominance is vital for multiple areas, including blood transfusions and organ transplants, where matching donor and recipient blood types can be critical. Recognizing the consequences of this inheritance type also helps in studying genetic diseases and anthropological research into population genetics.
Polygenic Inheritance
Polygenic inheritance refers to traits controlled by multiple genes, acting together to produce a phenotype. This is typical in complex traits such as skin color, height, and intelligence, where several genes contribute their effects cumulatively. As a result, the phenotypic outcome is continuous and shows a range of possibilities, unlike the discrete categories seen in Mendelian inheritance.
Considerations
Polygenic traits are of considerable significance in understanding how genetic variation is expressed within populations. It allows researchers to comprehend relationships between multiple genetic factors and how they interplay with environmental influences. This pattern is central to fields like quantitative genetics and plays a vital role in breeding programs in agriculture, especially when seeking to improve traits across generations.
Non-Mendelian inheritance broadens our understanding of genetic complexity, illustrating that genetics is not solely a matter of simple dominant or recessive alleles but involves intricate interactions among multiple factors.
Non-Mendelian inheritance broadens our understanding of genetic complexity, illustrating that genetics is not solely a matter of simple dominant or recessive alleles but involves intricate interactions among multiple factors.
In summary, non-Mendelian inheritance patterns elucidate the multifaceted nature of genetic expression, which is essential in fields from medicine to agriculture.
Genetic Mapping and Linkage Analysis
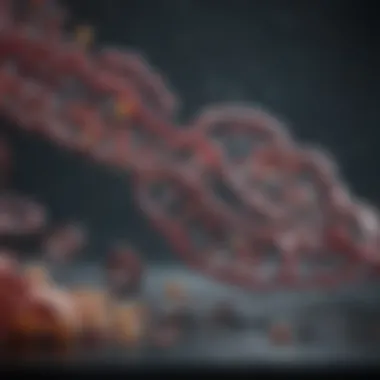
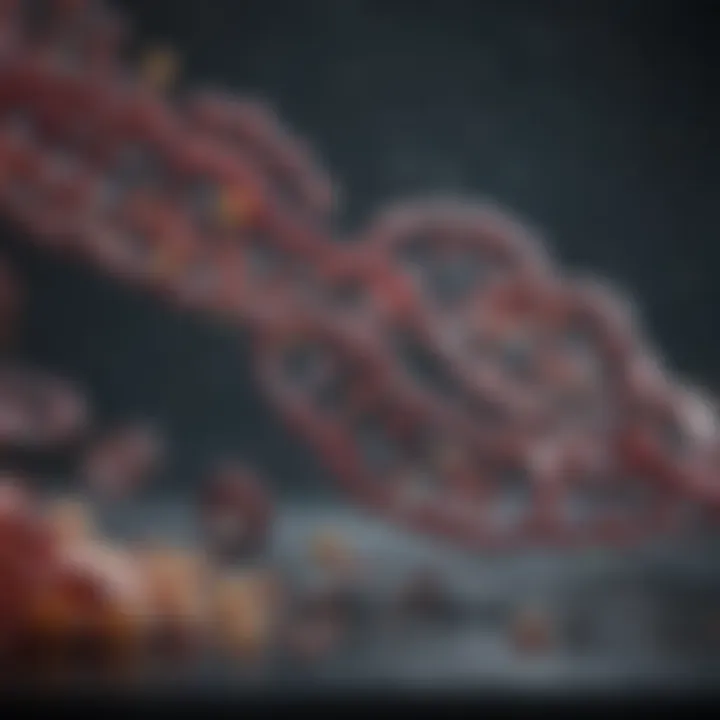
Genetic mapping and linkage analysis play a crucial role in understanding the hereditary patterns of genes and their relationships within the genome. By identifying the locations of genes on chromosomes and the distances between them, researchers can infer how traits are passed from one generation to the next. This knowledge holds invaluable implications across various fields, including medicine, agriculture, and evolutionary biology.
The process of genetic mapping helps in the identification of the genes associated with specific traits. These traits can be anything from disease resistance in plants to hereditary conditions in humans. Understanding the genetic basis of such traits enables scientists to develop effective strategies for breeding and gene therapy. Moreover, linkage analysis aids in determining whether certain genes are inherited together, thus revealing potential genetic interactions and dependencies.
Chromosomal Mapping Techniques
Chromosomal mapping techniques are essential for visualizing the structure of chromosomes and determining the positions of genes. One commonly utilized method is linkage mapping, which uses the frequency of recombination between genes to estimate the distance between them. The closer two genes are on a chromosome, the less likely they will be separated during cell division.
Another important technique is physical mapping, which utilizes tools such as fluorescence in situ hybridization (FISH) and next-generation sequencing. These methods allow researchers to pinpoint the exact locations of genes on chromosomes with high precision.
Key techniques include:
- Linkage Analysis: Assesses the inheritance patterns using family pedigrees to locate genes associated with certain traits.
- Single Nucleotide Polymorphism (SNP) Mapping: Exploits variations at a single base pair across the genome to determine gene locations.
- Whole Genome Sequencing (WGS): Provides a complete overview of an organism's genetic makeup, facilitating identification of genes and regulatory elements.
Determining Genetic Linkage
Determining genetic linkage involves assessing whether specific genes co-distributed more often than would be expected by chance alone. This can be accomplished through several methods, including statistical inference and molecular markers. Genetic linkage maps indicate how likely it is for genes to segregate together during meiosis, enhancing our understanding of genetic inheritance.
In practical applications, genetic linkage is valuable for
- Identifying Disease Genes: Researchers can focus on regions of chromosomes where linked genetic markers cluster, making it easier to locate genes responsible for genetic disorders.
- Crop Improvement: In agriculture, genetic linkage can assist in breeding programs that aim to improve desirable traits by identifying and selecting plants that carry specific linked alleles.
In summary, genetic mapping and linkage analysis provide essential insights into how genes relate to each other and contribute to various traits. These techniques offer essential tools for researchers looking to translate genetic knowledge into practical applications across disciplines.
Molecular Techniques in Transmission Genetics
Molecular techniques in transmission genetics form the backbone of modern genetic research. These methods allow scientists to manipulate, analyze, and understand genetic materials like DNA and RNA in intricate ways. The importance of these techniques lies in their capacity to elucidate the mechanisms of heredity and variation. By leveraging molecular tools, researchers can gain insights that extend far beyond traditional genetic approaches.
Advancements in molecular techniques have revolutionized various fields, including medicine, agriculture, and evolutionary biology. For students, researchers, and professionals, comprehending these techniques is vital, as they provide the foundation for exploring complex genetic questions and applications.
Applications of Genetic Engineering
Genetic engineering, a key application of molecular techniques, allows for the direct alteration of an organism's genome. This can involve adding, removing, or modifying specific genes to achieve desired traits. The applications are vast:
- Agriculture: Creating transgenic crops that are resistant to pests or have enhanced nutritional profiles.
- Medicine: Developing gene therapies for genetic disorders by correcting faulty genes.
- Biotechnology: Producing enzymes, antibiotics, or other bioactive compounds through modified organisms.
These applications showcase the transformative potential of genetic engineering, providing solutions to pressing global challenges.
CRISPR and Gene Editing
CRISPR technology represents a significant leap forward in the field of gene editing. Originally derived from a bacterial immune system, CRISPR allows for precise targeting and modification of genetic sequences. The advantages of using CRISPR include:
- Precision: Target specific locations in the genome without affecting surrounding genes.
- Efficiency: Faster and more cost-effective than traditional methods of gene editing.
- Flexibility: Applicable to many organisms, from plants to humans.
CRISPR is not without its challenges and ethical concerns. Issues related to off-target effects and the potential implications of germline editing are subjects of ongoing debate. However, its promise in areas such as disease treatment and agricultural advancement cannot be understated.
Genetic engineering and CRISPR technology are paving the way for unprecedented advancements in science, targeting genetic disorders and improving food security in a changing world.
Genetic engineering and CRISPR technology are paving the way for unprecedented advancements in science, targeting genetic disorders and improving food security in a changing world.
Transmission Genetics in Medicine
The intersection of transmission genetics and medicine has profound implications for diagnosis, treatment, and understanding of various health conditions. This area leverages genetic information to inform medical practices. By understanding how genetic traits are inherited, health professionals and researchers can predict susceptibilities to diseases and tailor interventions accordingly. This approach emphasizes a transition from a one-size-fits-all strategy to a more personalized approach, enhancing treatment effectiveness and patient outcomes.
Genetic Disorders and Their Inheritance
Genetic disorders are often the result of hereditary patterns, which indicate how these conditions pass through generations. Understanding these patterns can help identify individuals at risk or clarify the likelihood of disease presence in offspring. For instance, conditions like cystic fibrosis or sickle cell anemia follow Mendelian inheritance patterns, allowing for predictable probabilities of inheritance based on parental genotypes.
Some key points regarding genetic disorders include:
- Autosomal Dominant Disorders: Conditions such as Huntington's disease require only one copy of the mutated gene to manifest.
- Autosomal Recessive Disorders: Conditions like Tay-Sachs disease occur when an individual inherits two copies of a faulty gene, one from each parent.
- X-Linked Disorders: Disorders such as hemophilia are linked to genes on the X chromosome and show different inheritance patterns in males and females.
By exploring these inheritance patterns, healthcare providers can utilize genetic counseling to provide insights on the risks associated with specific disorders. This is particularly important for families with a history of genetic conditions, allowing them to make informed choices.
Personalized Medicine
Personalized medicine represents a significant shift in medical practice, as it incorporates genetic information to customize treatment plans for individual patients. By accounting for genetic differences among individuals, personalized medicine helps to achieve optimized therapeutic outcomes.
Key benefits of personalized medicine include:
- Tailored Treatment: Patients can receive medications and interventions that align closely with their genetic profile, increasing efficacy and reducing adverse effects.
- Predictive Approaches: Understanding an individual's genetic makeup allows for predictions about potential responses to treatments and the likelihood of side effects.
- Proactive Health Management: Early identification of genetic predispositions facilitates proactive health strategies, empowering patients with knowledge about their personal health risks.
"Understanding the genetic basis of diseases opens pathways to innovative treatments and prevention strategies, making personalized medicine a cornerstone of modern healthcare."
"Understanding the genetic basis of diseases opens pathways to innovative treatments and prevention strategies, making personalized medicine a cornerstone of modern healthcare."
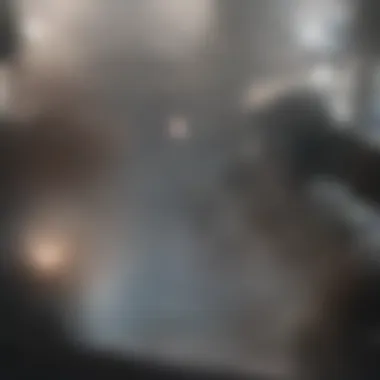
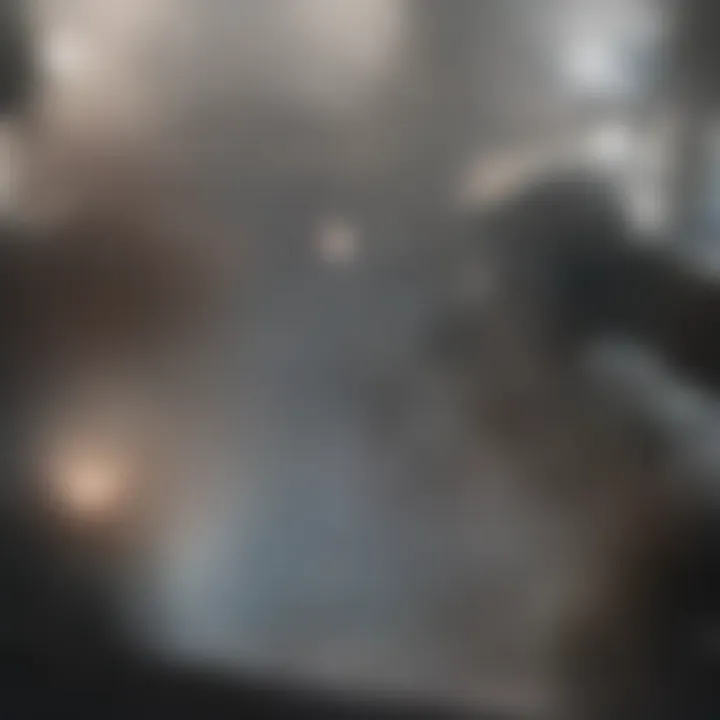
Agricultural Applications of Transmission Genetics
Agricultural applications of transmission genetics play a vital role in how we produce food and improve crop resilience, quality, and yield. As the global population continues to rise, there is increasing pressure on agricultural systems to meet food demands efficiently. This section explores the significance of transmission genetics in agriculture, focusing on two key areas: the development of transgenic crops and the enhancement of biodiversity for crop improvement.
Transgenic Crops
Transgenic crops are those that have been genetically modified to express specific traits not naturally found in the organism. This process typically involves the introduction of a gene from another organism into the plant's genetic material. The primary benefits of transgenic crops include:
- Increased Resistance to Pests and Diseases: Many genetically modified crops have been engineered to be resistant to pests and diseases, reducing the need for chemical pesticides. For instance, Bt corn has been designed to express a gene from the bacterium Bacillus thuringiensis. This gene produces a protein toxic to certain pests, making the crop safer and more sustainable.
- Enhanced Nutritional Value: Certain transgenic crops are engineered to contain higher levels of essential nutrients. Golden Rice, for example, has been modified to produce beta-carotene, which can help combat vitamin A deficiency in regions where rice is a staple food.
- Increased Yield: By enhancing traits such as drought tolerance, transgenic crops can thrive in less-than-ideal conditions. This ensures higher yields even in adverse climates, contributing to food security in challenging environments.
Nevertheless, the adoption of transgenic crops is not without controversy. Concerns around environmental impact, loss of biodiversity, and potential health risks are central to the debate on genetically modified organisms (GMOs). Regulatory frameworks therefore play an essential role in overseeing the development and use of transgenic crops in agriculture.
Biodiversity and Crop Improvement
The utilization of transmission genetics extends beyond transgenic crops. Biodiversity is critical for crop improvement and helps maintain ecosystem balance, encouraging sustainable agricultural practices. Here are some key points regarding biodiversity in crop improvement:
- Resilient Crop Varieties: Genetic diversity allows the development of crop varieties that can withstand environmental stresses such as drought, salinity, and disease. This resilience is crucial for adapting to climate change.
- Conservation of Traditional Varieties: Many traditional varieties of crops possess unique genes that can confer advantageous traits. By studying these varieties, scientists can identify valuable genetic traits that might be lost in commercial monocultures.
- Sustainable Practices: Biodiversity promotes sustainable agricultural practices. By employing methods that emphasize genetic diversity, farmers can reduce dependence on chemical inputs. Crop rotation, intercropping, and other techniques can enhance soil health and reduce pest outbreaks, leading to less environmental damage.
"Biodiversity is the key that unlocks the potential of our agricultural systems, ensuring food security for future generations."
"Biodiversity is the key that unlocks the potential of our agricultural systems, ensuring food security for future generations."
Ethical Considerations in Genetic Research
Ethical considerations in genetic research are paramount to ensuring responsible scientific practice. This topic encompasses the fairness, moral integrity, and potential societal implications of advancements in genetics. As genetic technologies evolve, the ramifications of their application require serious reflection. Researchers, clinicians, and policymakers must deliberate on how these technologies may affect individuals and communities alike.
The benefits of addressing these ethical considerations include fostering public trust, promoting responsible use of genetic information, and mitigating risks associated with genetic discrimination. If society does not take ethical implications into account, it can lead to misuse of genetic data, where individuals may face discrimination based on genetic predispositions. These reflections are vital in ensuring that genetic research serves to benefit humanity rather than harm it.
"Research in genetics must navigate a complex landscape of moral considerations if it is to yield positive outcomes."
"Research in genetics must navigate a complex landscape of moral considerations if it is to yield positive outcomes."
Ethics of Gene Editing
The ethics of gene editing forms a critical component of discussions around transmission genetics. With techniques like CRISPR, which allows for precise modification of genetic material, questions arise regarding the extent to which humans should alter life. Is it ethical to modify human embryos, for instance? Such actions could lead to unforeseen consequences in the gene pool. Decisions must consider not only the direct impacts on individuals but also broader ecological and societal ramifications.
Moreover, consent becomes a pivotal issue with gene editing. For example, can researchers truly obtain informed consent from future generations who will inherit edited genes? The potential for long-term effects raises the question of whether it is right to make changes that affect people who cannot consent at all. An ethical framework is necessary to guide these decisions, ensuring they align with societal values.
Regulatory Perspectives
Regulatory perspectives on genetic research and gene editing are crucial for guiding ethical practice. Various countries have different regulations, which reflect their cultural and ethical standards. Agencies such as the Food and Drug Administration (FDA) in the United States, or the European Medicines Agency (EMA) in Europe, play key roles in overseeing genetic research. These regulations aim to strike a balance between encouraging innovation and protecting public safety.
Policies must be dynamic, adapting to the rapid advancements in genetic technologies while ensuring rigorous oversight. They should address issues such as data confidentiality, consent, and equity in access to genetic innovations. By establishing clear regulatory frameworks, governments can help prevent genetic discrimination and ensure that research benefits society as a whole.
In summary, ethical considerations are vital for the responsible advancement of transmission genetics. They encompass the moral implications of gene editing and the necessity for regulatory accountability. These facets are essential for guiding the future of genetic research in a manner that respects human dignity and promotes equality.
Future Directions in Transmission Genetics
Transmission genetics is a dynamic and rapidly evolving field. As such, understanding its future directions is essential. The relevance of this topic in the broader context of genetics cannot be overstated. New technologies are transforming how genetic research is conducted, while interdisciplinary collaboration is opening novel pathways for discovery and innovation.
Emerging Technologies
The advent of cutting-edge technologies significantly enhances the capabilities of transmission genetics. One of the most prominent tools is CRISPR, a groundbreaking gene-editing technology that allows precise alterations to DNA sequences in various organisms. With its ease of use and efficiency, researchers are now exploring ways to apply CRISPR technology to understand gene function and inheritance patterns more thoroughly.
Furthermore, advancements in next-generation sequencing make it possible to analyze whole genomes rapidly. This technology facilitates comprehensive studies of genetic variation within populations, aiding in the identification of genetic markers linked to diseases or important traits. The accumulation of such genetic data can help researchers develop better models of inheritance and, ultimately, lead to improved agricultural and medical outcomes.
In addition, bioinformatics plays a critical role in analyzing the vast amounts of data generated through these technologies. By integrating computational tools with genetic research, scientists can uncover complex relationships and patterns that were previously difficult to detect. As these technologies advance, they will provide new insights into genetic transmission mechanisms, helping to refine the principles of inheritance.
Interdisciplinary Approaches
The future of transmission genetics lies not only in technology but also in collaborative efforts across disciplines. Bridging genetics with fields such as epigenetics, genomics, and phenomics can yield rich, multifaceted insights into heredity and variation.
For instance, epigenetic research focuses on how external factors influence gene expression. Combining this knowledge with traditional genetic principles can lead to an understanding of how environment interacts with genetic predisposition. This is crucial for comprehending complex traits and diseases.
Moreover, incorporating agriculture sciences and environmental studies allows for a holistic approach to genetic research. By understanding plant and animal genetics in relation to their environment, scientists can create more sustainable agricultural practices and improve food security.
The intersection of various scientific disciplines will be crucial for innovation in transmission genetics.
The intersection of various scientific disciplines will be crucial for innovation in transmission genetics.
As researchers continue to work across boundaries, novel treatments for genetic disorders and advances in crop improvement will emerge. This interdisciplinary approach not only enriches transmission genetics but also ensures the discipline remains relevant and impactful in addressing contemporary challenges.
The End
The conclusion serves as a crucial component in any scholarly work, synthesizing the content and guiding the reader towards significance. In this article, the conclusion encapsulates the core of transmission genetics, emphasizing its essential role in understanding heredity and biological variation. A clear understanding of these principles not only enhances academic insight but also underlines the applied dimensions of genetics in medicine, agriculture, and evolutionary studies.
Summarizing Key Insights
In reviewing the key points discussed throughout this article, it is evident that transmission genetics provides the foundation for modern genetic research. From Mendelian inheritance, which set the stage with its laws of segregation and independent assortment, to the advancements in molecular techniques such as CRISPR, each section contributes to a robust understanding of how genetic traits are passed through generations. The exploration of non-Mendelian inheritance patterns further illustrates the complexity of genetic interactions, revealing that the transmission of traits is not always straightforward. This depth of knowledge is critical for students, educators, and researchers who navigate this multifaceted field.
Importance of Transmission Genetics
The significance of transmission genetics cannot be overstated. It is vital for developing strategies in personalized medicine, where understanding an individual's genetic makeup leads to tailored treatments. In agriculture, the applications are equally profound, as genetic modifications help create resilient crops. Moreover, insights from transmission genetics pave the way for advancements in conservation biology, aiding in the preservation of biodiversity. Thus, the consideration of ethical implications in genetic research remains paramount, ensuring that progress benefits society while adhering to moral standards.